- Case report
- Open access
- Published: 26 March 2021
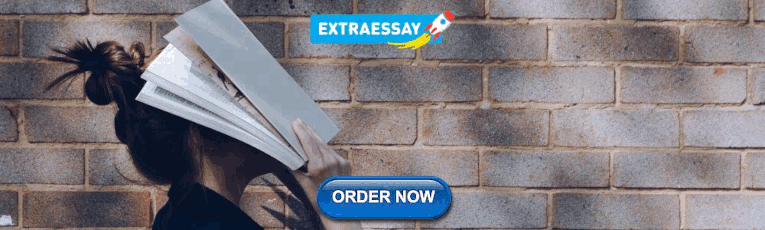
Coronavirus disease 2019 and dengue: two case reports
- Abdullah Isneen Hilmy 1 ,
- Rajib Kumar Dey 1 ,
- Hisham Ahmed Imad 2 , 3 ,
- Abdul Azeez Yoosuf 1 ,
- Ali Nazeem 1 &
- Ali Abdulla Latheef 1 , 4
Journal of Medical Case Reports volume 15 , Article number: 171 ( 2021 ) Cite this article
6950 Accesses
16 Citations
3 Altmetric
Metrics details
The pandemic of this century has overwhelmed the healthcare systems of affected countries, and all resources have been diverted to coronavirus disease 2019. At the onset, coronavirus disease 2019 can present as any other acute febrile undifferentiated illness. In tropical regions, clinicians are increasingly challenged to differentiate these febrile illnesses without the use of diagnostics. With this pandemic, many of these tropical diseases are neglected and go underreported. Dengue is holoendemic in the Maldives, and dengue viruses circulate throughout the year. Reports about coinfections with dengue virus and severe acute respiratory syndrome coronavirus 2 are scarce, and the outcome and the dynamics of the disease may be altered in the presence of coinfection. We have described the clinical manifestation and serial laboratory profile, and highlighted the atypical findings uncommon in dengue infection.
Case presentation
Case 1 was a 39-year old Asian male, presented on day 6 of dengue infection with warning signs. Reverse transcription polymerase chain reaction for severe acute respiratory syndrome coronavirus 2 that was done as per hospital protocol was found to be positive. Case 2 was a 38-year old Asian male, was admitted on day 5 of illness with symptoms of acute respiratory infection with positive reverse transcription polymerase chain reaction for severe acute respiratory syndrome coronavirus 2. Evaluation of progressive leukopenia and thrombocytopenia showed positive dengue serology.
Clinicians must be conscientious when working on the differential diagnosis of possible tropical diseases in cases of coronavirus disease 2019, specifically, when patients develop hemoconcentration, thrombocytopenia, and transaminitis with elevated expression of aspartate higher than alanine transaminase, which is frequently observed in dengue infection. Caution must be taken during the administration of intravenous fluids when treating patients with coronavirus disease 2019 and dengue coinfection, as coronavirus disease 2019 patients are more prone to develop pulmonary edema. Timely diagnosis and appropriate management are essential to avoid the devastating complications of severe forms of dengue infection. It is important to repeat and reconfirm the dengue serology in coronavirus disease 2019 patients to avoid false positivity. Diligence and care must be taken not to neglect other endemic tropical diseases in the region during the present pandemic.
Peer Review reports
Introduction
The coronavirus disease 2019 (COVID-19) pandemic began in late December 2019. Over 19 million confirmed cases and 700,000 fatalities have been reported. At present, only 12 countries worldwide have not been affected by COVID-19 [ 1 ]. The first case of COVID-19 in the Maldives was confirmed on 7 March 2020 [ 2 ]. Most of the confirmed cases were asymptomatic, and among the symptomatic cases a majority had mild to moderate disease and a minority were critical. To date, the Maldives has had over 4769 confirmed cases of COVID-19 and 19 fatalities [ 3 ]. Several arboviral infections and other tropical diseases are endemic to the Maldives [ 4 , 5 , 6 , 7 , 8 , 9 , 10 , 11 , 12 , 13 ]. Among these, the most commonly diagnosed is dengue infection because of the widespread use of commercially available serological rapid diagnostic test kits [ 14 ]. Annually, the Maldives experiences a peak in the number of reported cases during the dengue outbreaks, especially during the monsoons. Factors contributing to this are poor vector control due to congested urbanization and limited efforts to segregate accumulated waste, which are potential vector breeding grounds [ 15 ]. As the COVID-19 pandemic has been the prime focus of attention in the past 7 months, many of the existing problems such as dengue have been neglected. Severe forms of dengue if left undetected can be fatal [ 16 , 17 , 18 ]. These include dengue hemorrhagic fever, where in addition to bleeding, plasma leakage occurs and expanded dengue syndrome with multisystemic involvement. These classifications are now collectively referred to as severe dengue [ 19 ]. Many countries where dengue is endemic have expressed concerns over the devastating impact a dengue outbreak could have during the COVID-19 pandemic [ 20 , 21 , 22 ], while others express how dengue and COVID-19 share similarities in both clinical and laboratory findings [ 23 ]. One report demonstrated a false dengue serological test in a COVID-19 case [ 24 ]. There are also reports of serological overlap resulting in false positive dengue serology [ 25 ]. Reports about coinfections with dengue virus and severe acute respiratory syndrome coronavirus 2 (SARS-CoV-2) are scarce [ 24 , 26 ]. Herein, we describe the clinical manifestation and serial laboratory profile and highlight the atypical findings uncommon in dengue fever and dengue hemorrhagic fever when coinfected with SARS-CoV-2.
A 39-year-old, Asian male, who is a migrant worker presented to the emergency department with a history of persistent high-grade fever, retro-orbital headache, fatigue, and myalgia for the past 6 days. He also complained of right upper quadrant pain, vomiting, and loose stools. There was no history of rash, arthralgia, or bleeding. On physical examination, he was dehydrated and the vitals recorded were a body temperature of 37.5°C, heart rate of 80 beats per minute, blood pressure of 113/78 mmHg, pulse pressure of 35 mmHg, respiratory rate of 20 breaths per minute, capillary refill time of less than 2 seconds, and oxygen saturation of 98% in room air. Systemic examination was unremarkable except for right upper quadrant tenderness with a normal liver span. Bedside ultrasonography of the abdomen revealed gall bladder wall edema. The laboratory investigations at presentation and serial laboratory profiles are tabulated in Table 1 , which shows hemoconcentration of 19% and transaminitis. Dengue rapid test (SD BIOLINE Dengue DUO®) revealed a negative non-structural protein 1 (NS1) with a positive immunoglobulin (Ig)M and IgG. This was later reconfirmed with a positive dengue enzyme-linked immunosorbent assay (ELISA) IgM and IgG (Panbio ® Standard Diagnostics). Reverse transcription polymerase chain reaction (rRT-PCR) (Liferiver TM 2019-nCoV Real Time Multiplex RT-PCR Kit) for COVID-19 done as per hospital admission protocol was positive with a cycle threshold of 28.35. The patient was admitted to a COVID-19 facility and kept in isolation with a diagnosis of dengue hemorrhagic fever grade I with COVID-19. As the patient was vitally stable with a minimal increase in hemoconcentration, he was managed conservatively with oral fluids. On day 5 of illness, the patient developed non-productive cough and sore throat for which a chest X-ray was done and no abnormality was found. The patient was monitored daily to look for development of warning signs of dengue fever, including hemoconcentration. The patient had an uneventful hospital stay and was discharged after completing the mandatory isolation period of 14 days.
A 38-year-old Asian male presented with a history of intermittent fever and generalized headache for the past 5 days. He also complained of sore throat, dysgeusia, and anosmia for a duration of 3 days following the onset of fever. Being a close contact of a COVID-19 patient, he consulted an online clinic where he was referred to the hospital for evaluation. On examination, he appeared dehydrated, with a body temperature of 37.4°C, pulse rate of 84 beats per minute, blood pressure of 100/60 mmHg, pulse pressure of 40 mmHg, respiratory rate of 21 breaths per minute, capillary refill time of less than 2 seconds, and oxygen saturation of 97% in room air. Other systemic examination was unremarkable. With a positive rRT-PCR for SARS-CoV-2 (Ct value 24.45) he was admitted and kept in isolation. His laboratory parameters are tabulated in Table 2 , which shows leukopenia and thrombocytopenia. In view of the depleting trend of total leukocyte count and platelets, a dengue rapid test was requested, which showed a negative NS1 and positive IgM/IgG. This was reconfirmed with a positive dengue ELISA serology of anti-dengue IgM and IgG. Hence, the patient was diagnosed with COVID-19 with dengue fever. The patient had minimal hemoconcentration, and as he was able to take fluids adequately he was managed with oral fluids and monitored daily for the development of warning signs of dengue or worsening of the severity of COVID-19. The patient did not have any fever spikes after admission. The patient’s platelets gradually improved, and symptoms resolved over a period of 1 week. The patient was discharged after completing the 14-day mandatory period of isolation.
Here we describe two cases of coinfection of dengue fever and COVID-19. The first case had presented with symptoms of dengue fever, and after 5 days of admission he developed symptoms of COVID-19. The second case was admitted with mild COVID-19, and during the course of illness, in view of mild hemoconcentration and progressive decline in leukocyte and thrombocyte counts, he was tested positive for dengue fever. In coinfections, one virus can suppress or augment the other, leading to varying clinical manifestations of the diseases.
The COVID-19 pandemic has spread across the globe, including areas where other tropical diseases are endemic such as the Maldives [ 6 , 8 , 9 , 10 , 15 ]. Clinicians are challenged with the additional burden of possible coinfections with other tropical diseases that have the potential to complicate the course of illness [ 26 ]. Coinfections with COVID-19 have been reported with multiple bacteria and viruses [ 27 , 28 , 29 , 30 , 31 ]. Nevertheless, coinfection with dengue viruses and SARS-CoV-2 are still scarce, with only a few reports describing false-positive dengue serology [ 24 , 32 ]. The healthcare system of the Maldives is stretched and may not respond adequately to a dual outbreak because of limited manpower and infrastructure [ 33 ]. Every year, the Maldives experiences a dengue outbreak during the monsoons. From the year 2011, an average of 1543 cases have been reported annually [ 15 ]. In 2018, 3494 cases were reported, and in 2019, 5023 cases were reported, which was a record number for the Maldives [ 34 ]. Despite an expected increase in cases, so far only 225 cases have been reported, while dengue cases are on the rise in other endemic regions [ 34 , 35 ]. We postulate that healthcare providers are more focused on COVID-19, which has resulted in lower rates of testing or reporting of dengue virus. In addition, individuals with mild symptomatic dengue infections perhaps are not visiting hospitals because of the pandemic and lockdowns. COVID-19 may present as an undifferentiated acute febrile illness [ 36 ]. In one study, 87.9% of COVID-19 patients presented with fever, 67.7% presented with cough, and 13.7% of patients had headache [ 37 ]. Nevertheless, information regarding the frequency of fever in dengue infection is limited in most prospective studies, mostly due to confounders within the study designs where fever remains an inclusion criterion. Fever can be absent in the elderly population or, if present, can occur over 90% of the time [ 38 ]. Cough in dengue infection has been reported in 67% of cases, which can be due to pleural effusion observed during plasma leakage in dengue hemorrhagic fever (DHF) [ 39 , 40 ]. Headache in dengue infection has been reported in 89% of cases and more commonly in DHF [ 41 ]. Bleeding in COVID-19 has been reported to be associated with morbidity, but frequency of bleeding manifestations in COVID-19 remains low [ 42 ]. In contrast, bleeding in dengue infection occurs more frequently and is associated with increasing severity [ 41 ]. The hematological profile in COVID-19 and dengue infection are similar. Neutrophils are increased early in both infections with leukopenia and thrombocytopenia. The discrepancy in the hematological profile in both infections is lymphopenia, which is observed in COVID-19 during the course of illness in contrast to a reverse neutrophil-to-lymphocyte ratio in dengue infection. Transaminitis is observed in both infections; however, the expression of aspartate aminotransferase is greater than alanine transaminase in dengue infection [ 43 , 44 ]. Our first case was initially diagnosed as dengue fever with warning signs, and COVID-19 was detected during the screening process before admission [ 45 ]. On day 5 of admission, he developed a non-productive cough and sore throat that could be attributed to COVID-19. The cough could also be due to dengue-related pleural effusion, which was not evident on his chest X-ray in posterior–anterior view. However, a chest X-ray in lateral decubitus view is more sensitive to detect minimal effusion [ 46 ]. In our second case, the patient was admitted with a diagnosis of mild COVID-19. With a depleting trend of leukocytes and platelets, dengue serology was performed, which was positive. Case 1 had a hemoconcentration of 19% and gall bladder wall edema suggestive of plasma leakage [ 47 ]. As there was no bleeding manifestation, this case was classified as DHF grade 1 [ 48 ]. In case 1, liver biochemistry revealed an atypical profile with alanine transaminase expression greater than that of aspartate aminotransferase, which may be due to coinfection. However, initially in case 2, we observed mild transaminitis with an elevation of aspartate aminotransferase expression compared with that of alanine transaminase, which is consistent with dengue infection. Case 2 had leukopenia, which may be seen in both infections, which started to improve on day 3 of admission. In both cases, NS1 was negative, which could have been due to the late presentation. Treatment was mainly symptomatic. Intravenous fluid was avoided as COVID-19 patients are more prone to develop pulmonary edema [ 49 ]. Coinfections of two viruses can reduce or augment disease severity [ 50 ]. The most common outcome is viral interference, where one virus competitively suppresses the replication of the other [ 51 ]. This could have played an important role in changing the dynamics of dengue virus infection and the natural history of the disease. This could explain the two cases failing to demonstrate the typical features of dengue infection, both in its disease progression and the laboratory findings. More data are required to determine the changing dynamics of coinfection of the dengue virus with SARS-CoV-2. Furthermore, clinicians should use commercially available dengue serology judiciously with growing evidence of cross-reaction with other flaviviruses [ 52 ]. Limitations in this report include the inability to utilize the gold standard to diagnose dengue infection either by virus isolation or RT-PCR. In addition to this, dengue serotyping was also not done, which could have provided valuable information regarding virulence and disease dynamics.
During this pandemic, it is important to consider other tropical diseases that are endemic to the tropics, such as dengue, Zika, chikungunya infections, or scrub typhus. Coinfection with two viruses may change the dynamics and natural history of disease progression, which may result in atypical presentation of dengue infection and COVID-19. In cases of COVID-19 with atypical presentations, such as increased hematocrit, depleted platelets, and transaminitis with a greater expression of aspartate aminotransferase than of alanine transaminase, dengue infection should be considered. In COVID-19 patients with positive dengue serology, it is important to repeat and reconfirm the test to avoid false positivity. It is important to keep an open mind when treating patients with COVID-19 and be vigilant of atypical signs and symptoms that may indicate any other tropical infection. Clinicians should be cautious in giving intravenous fluids when treating patients with COVID-19 and dengue coinfection, as COVID-19 patients are more prone to develop pulmonary edema.
Availability of data and materials
Not applicable.
University JH. COVID-19 Dashboard by the Center for Systems Science and Engineering (CSSE) at Johns Hopkins University (JHU)
Health Mo. First cases of COVID-19 confirmed in the Maldives. 2020.
Agency HP. COVID-19 Statistics Dashboard.
Trojanek M, Tomickova D, Rohacova H, Kosina P, Gebousky J, Dvorak J, et al. Dengue fever cases in Czech workers returning from the Maldives. Epidemiol Mikrobiol Imunol. 2013;62:100–5.
PubMed Google Scholar
Mutoh Y, Moriya A, Yasui Y, Saito N, Takasaki T, Hiramatsu S, et al. Two cases of dengue virus type 2 (DENV-2) infection in a Japanese couple returning from the Maldives during the 2018 dengue outbreak. Jpn J Infect Dis. 2020;73:58–60.
Article Google Scholar
Korhonen EM, Huhtamo E, Smura T, Kallio-Kokko H, Raassina M, Vapalahti O. Zika virus infection in a traveller returning from the Maldives, June 2015. Euro Surveill. 2016;21:99.
Yoosuf AA, Shiham I, Mohamed AJ, Ali G, Luna JM, Pandav R, et al. First report of chikungunya from the Maldives. Trans R Soc Trop Med Hyg. 2009;103:192–6.
Dudouet P, Gautret P, Larsen CS, Diaz-Menendez M, Trigo E, von Sonnenburg F, et al. Chikungunya resurgence in the Maldives and risk for importation via tourists to Europe in 2019–2020: a GeoSentinel case series. Travel Med Infect Dis. 2020;9:101814.
Imad HA, Riza S, Reesha F, Ali A, Muaz M, Sujau I, et al. Severe typhoid in Maldives. 11th International Conference on Typhoid and other Invasive Salmonelloses. 2019.
Imad HA, Tanyaratsrisakul S, Piyaphanee W, Wattanagoon Y. Skin lesion from Maldives: classic but forgotten. Travel Med Infect Dis. 2017;17:74–5.
Receveur M, Ezzedine K, Pistone T, Malvy D. Chikungunya infection in a French traveller returning from the Maldives, October 2009. Euro Surveill. 2010;15:19494.
Article CAS Google Scholar
Pfeffer M, Hanus I, Loscher T, Homeier T, Dobler G. Chikungunya fever in two German tourists returning from the Maldives, September 2009. Euro Surveill. 2010;15:9.
Google Scholar
Lewis MD, Yousuf AA, Lerdthusnee K, Razee A, Chandranoi K, Jones JW. Scrub typhus reemergence in the Maldives. Emerg Infect Dis. 2003;9:1638–41.
Abdulla AA, Rasheeda F, Ahmed IN, Aboobakur M. An evaluation of the surveillance system for dengue virus infections in Maldives. WHO South East Asia J Public Health. 2014;3:60–8.
Bangert M, Latheef AT, Dev Pant S, Nishan Ahmed I, Saleem S, Nazla Rafeeq F, et al. Economic analysis of dengue prevention and case management in the Maldives. PLoS Negl Trop Dis. 2018;12:e0006796.
Laoprasopwattana K, Chaimongkol W, Pruekprasert P, Geater A. Acute respiratory failure and active bleeding are the important fatality predictive factors for severe dengue viral infection. PLoS ONE. 2014;9:e114499.
Wei HY, Shu PY, Hung MN. Characteristics and risk factors for fatality in patients with dengue hemorrhagic fever, Taiwan, 2014. Am J Trop Med Hyg. 2016;95:322–7.
Magpusao NS, Monteclar A, Deen JL. Slow improvement of clinically-diagnosed dengue haemorrhagic fever case fatality rates. Trop Doct. 2003;33:156–9.
Wiwanitkit V. Accuracy and applicability of the revised WHO classification (2009) of dengue. Infection. 2013;41:1047.
Wu D, Lu J, Liu Q, Ma X, He W. To alert coinfection of COVID-19 and dengue virus in developing countries in the dengue-endemic area. Infect Control Hosp Epidemiol. 2020;22:1.
Saavedra-Velasco M, Chiara-Chilet C, Pichardo-Rodriguez R, Grandez-Urbina A. Inga-Berrospi F [Coinfection between dengue and covid-19: need for approach in endemic zones.]. Rev Fac Cien Med Univ Nac Cordoba. 2020;77:52–4.
Miah MA, Husna A. Coinfection, coepidemics of COVID-19, and dengue in dengue-endemic countries: a serious health concern. J Med Virol. 2020;8:22.
Henrina H, Putra I, Lawrensia S, Handoyono Q, Alius C. Coronavirus disease of 2019: a mimicker of dengue infection? SN Comprehensive Clinical Medicine 2020.
Yan G, Lee CK, Lam LTM, Yan B, Chua YX, Lim AYN, et al. Covert COVID-19 and false-positive dengue serology in Singapore. Lancet Infect Dis. 2020;20:536.
Kembuan GJ. Dengue serology in Indonesian COVID-19 patients: coinfection or serological overlap? IDCases. 2020;22:e00927.
Tun SZ. Thailand records first coronavirus death: health official. REUTERS. 2020.
Toombs JM, Van den Abbeele K, Democratis J, Mandal AKJ, Missouris CG. Pneumococcal coinfection in COVID-19 patients. J Med Virol. 2020.
Ozaras R, Cirpin R, Duran A, Duman H, Arslan O, Bakcan Y, et al. Influenza and COVID-19 coinfection: report of six cases and review of the literature. J Med Virol. 2020.
Menon AA, Berg DD, Brea EJ, Deutsch AJ, Kidia KK, Thurber EG, et al. A case of COVID-19 and Pneumocystis jirovecii coinfection. Am J Respir Crit Care Med. 2020;202:136–8.
Khaddour K, Sikora A, Tahir N, Nepomuceno D, Huang T. Case report: the importance of novel coronavirus disease (COVID-19) and coinfection with other respiratory pathogens in the current pandemic. Am J Trop Med Hyg. 2020;102:1208–9.
Fan BE, Lim KGE, Chong VCL, Chan SSW, Ong KH, Kuperan P. COVID-19 and Mycoplasma pneumoniae coinfection. Am J Hematol. 2020;95:723–4.
Joob B, Wiwanitkit V. COVID-19 can present with a rash and be mistaken for dengue. J Am Acad Dermatol. 2020;82:e177.
Maldives U. Addressing the socio-economic impact of COVID-19 on the Maldives 2020.
Health Mo. Monthly Communicable Disease Report. 2020.
Control ECfDPa. Dengue worldwide overview. 2020.
Nunthavichitra S, Prapaso S, Luvira V, Muangnoicharoen S, Leaungwutiwong P, Piyaphanee W. Case report: COVID-19 presenting as acute undifferentiated febrile illness—a tropical world threat. Am J Trop Med Hyg. 2020;103:83–5.
Guan WJ, Ni ZY, Hu Y, Liang WH, Ou CQ, He JX, et al. Clinical characteristics of coronavirus disease 2019 in China. N Engl J Med. 2020;382:1708–20.
Rowe EK, Leo YS, Wong JG, Thein TL, Gan VC, Lee LK, et al. Challenges in dengue fever in the elderly: atypical presentation and risk of severe dengue and hospital-acquired infection [corrected]. PLoS Negl Trop Dis. 2014;8:e2777.
Restrepo BN, Beatty ME, Goez Y, Ramirez RE, Letson GW, Diaz FJ, et al. Frequency and clinical manifestations of dengue in urban Medellin. Colombia J Trop Med. 2014;2014:872608.
Marchiori E, Hochhegger B, Zanetti G. Pulmonary manifestations of dengue. J Bras Pneumol. 2020;46:e20190246.
Imad HA, Phumratanaprapin W, Phonrat B, Chotivanich K, Charunwatthana P, Muangnoicharoen S, et al. Cytokine expression in dengue fever and dengue hemorrhagic fever patients with bleeding and severe hepatitis. Am J Trop Med Hyg. 2020;102:943–50.
Al-Samkari H, Karp Leaf RS, Dzik WH, Carlson JCT, Fogerty AE, Waheed A, et al. COVID-19 and coagulation: bleeding and thrombotic manifestations of SARS-CoV-2 infection. Blood. 2020;136:489–500.
Samanta J, Sharma V. Dengue and its effects on liver. World J Clin Cases. 2015;3:125–31.
Ong J, Young BE, Ong S. COVID-19 in gastroenterology: a clinical perspective. Gut. 2020;69:1144–5.
Organization WH. Handbook for clinical management of dengue, WHO and Special Programme for Research and Training in Tropical Diseases (TDR) report. 2012.
Kalayanarooj S. Clinical manifestations and management of dengue/DHF/DSS. Trop Med Health. 2011;39:83–7.
Tavares MA, Joao GAP, Bastos MS, Gimaque JBL, Almeida ACG, Ngo TT, et al. Clinical relevance of gallbladder wall thickening for dengue severity: a cross-sectional study. PLoS ONE. 2019;14:e0218939.
Hadinegoro SR. The revised WHO dengue case classification: does the system need to be modified? Paediatr Int Child Health. 2012;32(Suppl 1):33–8.
Dagens A, Sigfrid L, Cai E, Lipworth S, Cheng V, Harris E, et al. Scope, quality, and inclusivity of clinical guidelines produced early in the covid-19 pandemic: rapid review. BMJ. 2020;369:m1936.
Diaz-Munoz SL. Viral coinfection is shaped by host ecology and virus-virus interactions across diverse microbial taxa and environments. Virus Evol. 2017;3:vex011.
Kumar N, Sharma S, Barua S, Tripathi BN, Rouse BT. Virological and immunological outcomes of coinfections. Clin Microbiol Rev. 2018;31:23.
Suzuki K, Nakayama EE, Saito A, Egawa A, Sato T, Phadungsombat J, et al. Evaluation of novel rapid detection kits for dengue virus NS1 antigen in Dhaka, Bangladesh, in 2017. Virol J. 2019;16:102.
Download references
Acknowledgements
We would like to express our sincere appreciation to all the front-liners across the globe for their bravery and dedication in combating this unprecedented event in our present lifetime. In addition, we would like to thank all the staff working at Indira Gandhi Memorial Hospital and the COVID-19 facility, including everyone at the multiagency taskforce overseeing the management of the current pandemic in the Maldives.
There was no financial support for this clinical case report.
Author information
Authors and affiliations.
Department of Internal Medicine, Indira Gandhi Memorial Hospital, Male’ 20002, Maldives
Abdullah Isneen Hilmy, Rajib Kumar Dey, Abdul Azeez Yoosuf, Ali Nazeem & Ali Abdulla Latheef
Mahidol-Osaka Center for Infectious Diseases, Faculty of Tropical Medicine, Mahidol University, Bangkok 10400, Thailand
Hisham Ahmed Imad
Department of Viral Infections, Research Institute for Microbial Diseases, Osaka University, Osaka 565-0871, Japan
Technical Advisory Group, Health Emergency Operation Center, Male’ 20002, Maldives
Ali Abdulla Latheef
You can also search for this author in PubMed Google Scholar
Contributions
AIH: conceptualization, writing original draft, data collection; RKD: writing review and editing, data collection; HAI: writing review and editing; AN: review and validation; AAY: review and validation; AAL: supervision, review and validation. All authors read and approved the final manuscript.
Corresponding author
Correspondence to Abdullah Isneen Hilmy .
Ethics declarations
Ethics approval and consent to participate, consent for publication.
Written informed consent was obtained from the patients for publication of this case report and any accompanying images. A copy of the written consent is available for review by the Editor-in-Chief of this journal.
Competing interests
The authors declare that they have no competing interests.
Additional information
Publisher’s note.
Springer Nature remains neutral with regard to jurisdictional claims in published maps and institutional affiliations.
Rights and permissions
Open Access This article is licensed under a Creative Commons Attribution 4.0 International License, which permits use, sharing, adaptation, distribution and reproduction in any medium or format, as long as you give appropriate credit to the original author(s) and the source, provide a link to the Creative Commons licence, and indicate if changes were made. The images or other third party material in this article are included in the article's Creative Commons licence, unless indicated otherwise in a credit line to the material. If material is not included in the article's Creative Commons licence and your intended use is not permitted by statutory regulation or exceeds the permitted use, you will need to obtain permission directly from the copyright holder. To view a copy of this licence, visit http://creativecommons.org/licenses/by/4.0/ . The Creative Commons Public Domain Dedication waiver ( http://creativecommons.org/publicdomain/zero/1.0/ ) applies to the data made available in this article, unless otherwise stated in a credit line to the data.
Reprints and permissions
About this article
Cite this article.
Hilmy, A.I., Dey, R.K., Imad, H.A. et al. Coronavirus disease 2019 and dengue: two case reports. J Med Case Reports 15 , 171 (2021). https://doi.org/10.1186/s13256-021-02707-7
Download citation
Received : 06 September 2020
Accepted : 28 January 2021
Published : 26 March 2021
DOI : https://doi.org/10.1186/s13256-021-02707-7
Share this article
Anyone you share the following link with will be able to read this content:
Sorry, a shareable link is not currently available for this article.
Provided by the Springer Nature SharedIt content-sharing initiative
- Coinfection
Journal of Medical Case Reports
ISSN: 1752-1947
- Submission enquiries: Access here and click Contact Us
- General enquiries: [email protected]
A case presentation of a fatal dengue myocarditis showing evidence for dengue virus-induced lesion
Affiliation.
- 1 University of São Paulo Medical School at Ribeirão Preto, São Paulo, Brazil.
- PMID: 24222821
- PMCID: PMC3821802
- DOI: 10.1177/2048872613475889
Dengue is a prevalent arthropod-borne viral disease in tropical and subtropical areas of the globe. Dengue clinical manifestations include asymptomatic infections; undifferentiated fever; dengue fever, which is characterized by fever, headache, retroorbital pain, myalgia, and arthralgia; and a severe form of the disease denominated dengue haemorrhagic fever/dengue shock syndrome, characterized by haemoconcentration, thrombocytopenia, and bleeding tendency. However, atypical manifestations, such as liver, central nervous system, and cardiac involvement, have been increasingly reported. We report an atypical and rare presentation of dengue disease marked by a dramatic and fatal cardiogenic shock due to acute myocarditis. Histopathological analysis of heart tissue showed several multifocal areas of muscle necrosis and intense interstitial oedema associated with clusters of virus particles inside the cardiomyocytes and in the interstitial space, providing evidence of a possible direct action of dengue virus on myocardium.
Keywords: Acute heart failure; acute myocarditis; cardiogenic shock; dengue fever.
Publication types
- Case Reports
- Fatal Outcome
- Myocarditis / virology*
- Severe Dengue*
- Shock, Cardiogenic / virology*
- Search Menu
- Advance Articles
- Editor's Choice
- Supplements
- Spot the Diagnosis
- Biomarker Spotlight
- ESC Journals App
- ESC Content Collections
- Author Guidelines
- Submission Site
- Why publish with EHJ-ACVC?
- Open Access Options
- Read & Publish
- Author Resources
- Self-Archiving Policy
- About European Heart Journal - Acute Cardiovascular Care
- About European Society of Cardiology
- ESC Publications
- Editorial Board
- Advertising & Corporate Services
- Developing Countries Initiative
- Journals on Oxford Academic
- Books on Oxford Academic
Article Contents
Introduction, case report, conclusions, conflict of interest.
- < Previous
A case presentation of a fatal dengue myocarditis showing evidence for dengue virus-induced lesion
- Article contents
- Figures & tables
- Supplementary Data
Carlos Henrique Miranda, Marcos de Carvalho Borges, André Schmidt, Antônio Pazin-Filho, Marcos Antônio Rossi, Simone Gusmão Ramos, Benedito Antônio Lopes da Fonseca, A case presentation of a fatal dengue myocarditis showing evidence for dengue virus-induced lesion, European Heart Journal. Acute Cardiovascular Care , Volume 2, Issue 2, 1 June 2013, Pages 127–130, https://doi.org/10.1177/2048872613475889
- Permissions Icon Permissions
Dengue is a prevalent arthropod-borne viral disease in tropical and subtropical areas of the globe. Dengue clinical manifestations include asymptomatic infections; undifferentiated fever; dengue fever, which is characterized by fever, headache, retroorbital pain, myalgia, and arthralgia; and a severe form of the disease denominated dengue haemorrhagic fever/dengue shock syndrome, characterized by haemoconcentration, thrombocytopenia, and bleeding tendency. However, atypical manifestations, such as liver, central nervous system, and cardiac involvement, have been increasingly reported. We report an atypical and rare presentation of dengue disease marked by a dramatic and fatal cardiogenic shock due to acute myocarditis. Histopathological analysis of heart tissue showed several multifocal areas of muscle necrosis and intense interstitial oedema associated with clusters of virus particles inside the cardiomyocytes and in the interstitial space, providing evidence of a possible direct action of dengue virus on myocardium.
Dengue, an arthropod-borne viral infection of humans, is endemic to tropical and subtropical regions of the world and represents an important public health problem. Dengue viruses are transmitted by the bite of the Aedes aegypti mosquito infected by the one of the four dengue virus serotypes: dengue-1, -2, -3, and -4. More recently, dengue disease has spread geographically to many previously unaffected areas and, as travelling around the world has become more accessible, physicians in temperate areas are more likely to see returning travellers with dengue infection. 1 , 12
Clinical manifestations include fever, headache, retro-orbital pain, rash, severe myalgia, and arthralgia. A more severe clinical presentation, dengue haemorrhagic fever/dengue shock syndrome (DHF/DSS), is characterized by increased vascular permeability, thrombocytopenia (platelets <100,000), bleeding tendency, and, in a small percentage of patients, circulatory shock. 2 – 5
In addition, especially during large outbreaks, atypical clinical manifestations have been described, such as encephalitis, Guillain-Barré syndrome, and fulminant hepatitis. 6 , 7
Cardiac involvement in dengue has been reported in few studies, usually resulting in a benign and self-limited disease. Although reports of a more severe disease with progression to cardiogenic shock and death have been increasingly described, 8 – 10 the pathogenesis of myocardial lesions has not been elucidated. We present a rare case of a fulminant and fatal myocarditis caused by dengue virus and provide more detailed histological evidence for a possible direct action of dengue virus on myocardial fibres.
A 37-year-old woman was admitted to the Emergency Department of the Clinical Hospital of the São Paulo University of Medical School at Ribeirão Preto with a history of progressive dyspnoea in the last 3 days that had recently evolved to dyspnoea at rest and orthopnoea, atypical chest pain, dizziness, and one episode of syncope. Eight days prior the admission, she complained of fever, headache, retro-orbital pain, arthralgia, and weakness. A dengue diagnosis was performed based on clinical and epidemiological grounds. Her past medical history was positive only for a diagnosis of Alport syndrome.
On clinical examination, she was in severe condition, agitated, with signs of poor peripheral perfusion, such as cold extremities and cyanosis. Heart rate was 145 bpm and blood pressure unrecordable. Cardiac and pulmonary auscultations were normal. Haemorrhagic suffusions or other skin lesions were absent. The 12-lead electrocardiogram detected atrial fibrillation, low voltage QRS, and diffuse ST-segment elevation.
Fluid resuscitation with 0.9% saline solution, infusion of sodium bicarbonate, and vasoactive drugs were promptly initiated. Dopamine was used in progressive doses up to 20 µg/kg/min, followed by increasing doses of norepinephrine up to 2 µg/kg/min, but haemodynamic stabilization was not achieved at any time.
Complementary exams are shown in Table 1 . Emergency transthoracic echocardiography was performed at bedside and showed mild pericardial effusion, without signs of cardiac tamponade, and severe left ventricular dysfunction with diffuse hypokinesia of left ventricular wall.
Laboratory test results obtained during hospital admission
Due to respiratory function deterioration, tracheal intubation and mechanical ventilation were initiated but she rapidly progressed to cardiopulmonary arrest with a pulseless electrical activity rhythm. Cardiopulmonary resuscitation was performed without success and the patient died 2 hours after hospital admission. Laboratory results obtained in the next days showed that real-time PCR results for Neisseria meningitidis, Streptococcus pneumoniae, Haemophilus influenzae , and seasonal and H1N1 influenza virus were negative. Serology for dengue (IgG and IgM ELISA) was positive and confirmed dengue disease.
Necroscopic examination revealed a dilated and flabby heart. Histopathological study showed marked interstitial oedema with a diffuse inflammatory infiltrate mainly composed of lymphomononuclear cells and fibroblasts. Diffuse foci of myocytolytic necrosis where neutrophils could be identified in association with mononuclear cells. There were no atherothrombotic lesions of main coronary or intramyocardial coronary vessels. There was no evidence of bacterial or fungal infection in any organs. Electron microscopic study of the myocardium disclosed clusters of virus particles in diffuse foci of cardiomyocytes, presenting dissolution of myofilaments, and in the interstitial space ( Figure 1 ).
(A) Representative image of the myocardium showing diffuse foci of myocytolytic necrosis stained in blue (Masson’s trichrome stain, bar 100 µm). (B and C) Detail of a focus of myocytolytic necrosis as seen with Masson’s trichrome staining (B) and haematoxylin and eosin staining (C). In C, the presence of neutrophils can be clearly disclosed beside mononuclear cell (bars 50 µm). (D) Representative image of electron microscopic findings showing cluster of dengue-like virus particle inside the cells and in the interstitial space. The dissolution of myofilaments is a prominent feature in cardiomyocytes (bar 2 µm). (E) Higher magnification showing a cluster of dengue-like virus particles (bar 500 nm) and dengue-like virus particles in detail (inset, bar 100 nm). The loss of virus particles depends on the fact that the myocardial tissue was first fixed in formaldehyde and processed for light microscope and reprocessed for electron microscopy
Dengue is a worldwide public health problem and causes innumerous deaths. More than 40% of the world’s population lives in dengue endemic areas, and the World Health Organization estimates that about 2.5 billion people in 100 countries are at risk of infection and that as many as 100 million people are infected by dengue viruses every year. In the majority of infected people, dengue is an auto-limited disease that resolves in 5–7 days. However, approximately 500,000 people develop a severe form, leading to about 20,000 deaths annually. Consequently, approximately 0.5% of dengue patients develops a severe form and requires a specialized treatment. 11 , 12
There are few reports of adult patients with acute heart failure during dengue virus infection, and, in two of them, this complication was considered to be the cause of death. 6 , 13 , 14 We report a rare case of a 37-year-old woman with dengue that developed a fulminant and fatal cardiogenic shock 8 days after disease onset and provided detailed histological evidence that support the hypothesis of a possible direct viral lesion on myocardial fibres.
The presence of IgM and IgG antibodies on the 8th day of disease and the absence of a medical appointment in any of the city health centres in the last 8 months indicate that this patient might have experienced a secondary dengue infection. The observation that complications are more frequent during secondary infection is well known.
Cardiac involvement in dengue and its pathogeneses have been seldom described and poorly investigated. In one study in Sri Lanka, 25% of dengue patients presented with one or more elevated markers of myocardial injury, such as increase in myoglobin, CK-MB, troponin T, N-terminal type B peptide, and/or heart-type fatty acid binding protein levels. 15 In another report of 102 children with DHF, 10 patients had acute myocarditis requiring use of inotropic drugs and one child died. 16 Studying 17 DHF/DSS patients with radionuclide ventriculography, Wali et al. 17 showed that seven patients had ejection fraction less than 40%, 12 had global hipokinesia, and, after 3 weeks of follow up, all alterations had returned to normal. Weerakoon et al. 18 performed autopsies on five patients who died due to dengue complications and showed histopathological evidence of myocarditis.
Cardiac arrhythmias are other clinical manifestations of myocarditis. Various arrhythmias have been described during dengue virus infection such as atrial fibrillation, ventricular tachycardia and even atrioventricular blocks. These arrhythmias are associated to syncope and even sudden death. 13 , 14 , 19 – 21 Our patient presented with atrial fibrillation at admission, which could have contributed to the cardiogenic shock and death.
The mechanism of myocardial damage in dengue could be the release of inflammatory mediators and/or the direct action of the virus on cardiomyocytes, as seen in acute myocarditis caused by other viruses. 22 Salgado et al., 23 using immunofluorescence confocal microscopy in heart tissue, reported that myotubes were infected by dengue virus in one child with fatal DHF, although the myocardium sections appeared morphologically normal, with minimal cellular infiltrates. Moreover, clinical characterization of myocarditis in this case was not complete.
We have demonstrated that the fulminant course of clinical dengue myocarditis was associated with intense interstitial oedema, several multifocal areas of necrosis, and diffuse inflammatory infiltration. Interestingly, the myocytolitic necrotic areas were replete with virus particles, therefore providing detailed histological evidence of a possible dengue direct action in cardiomyocytes. Further clinical and experimental studies are necessary to better understand the molecular mechanism of dengue virus-induced lesions on the myocardium.
Other pathogens occurring simultaneously or following dengue infection have been described. 24 , 25 In this case, infection by other pathogens were ruled out clinically and with laboratorial and histopathological data. We did not objectively exclude coxsackie B and other virus infections, but the temporal association with a dengue-like disease and the positive serology for dengue confirm dengue virus as the more probable causative agent in this patient.
Dengue virus can produce atypical manifestations as acute myocarditis leading to cardiogenic shock and death by a possible direct virus action on cardiomyocytes. Physicians taking care of dengue patients should be aware of this possible complication.
This research received no specific grant from any funding agency in the public, commercial or not-for-profit sectors.
Lupi O . Mosquito-borne hemorrhagic fevers . Dermatol Clin 2011 ; 29 : 33 – 38 .
Google Scholar
da Fonseca B A , Fonseca S N . Dengue virus infections . Curr Opin Pediatr 2002 ; 14 : 67 – 71 .
Deen J L , Harris E , Wills B et al. . The WHO dengue classification and case definitions: time for a reassessment . Lancet 2006 ; 368 : 170 – 173 .
Malavige G N , Fernando S , Fernando D J et al. . Dengue viral infections . Postgrad Med J 2004 ; 80 : 588 – 601 .
Teixeira M G , Barreto M L . Diagnosis and management of dengue . BMJ 2009 ; 339 : b4338 .
Gulati S , Maheshwari A . Atypical manifestations of dengue . Trop Med Int Health 2007 ; 12 : 1087 – 1095 .
Thomas L , Brouste Y , Najioullah F et al. . Prospective and descriptive study of adult dengue cases in an emergency department, in Martinique . Med Mal Infect 2009 ; 40 : 480 – 489 .
Kularatne S A , Pathirage M M , Medagama U A et al. . Myocarditis in three patients with dengue virus type DEN 3 infection . Ceylon Med J 2006 ; 51 : 75 – 76 .
Nagaratnam N , Siripala K , de Silva N . Arbovirus (dengue type) as a cause of acute myocarditis and pericarditis . Br Heart J 1973 ; 35 : 204 – 206 .
Obeyesekere I , Hermon Y . Arbovirus heart disease: myocarditis and cardiomyopathy following dengue and chikungunya fever – a follow-up study . Am Heart J 1973 ; 85 : 186 – 194 .
Pinheiro F P , Corber S J . Global situation of dengue and dengue haemorrhagic fever, and its emergence in the Americas . World Health Stat Q 1997 ; 50 : 161 – 169 .
Simmons C P , Farrar J J , Nguyen V et al. . Dengue . N Engl J Med 2012 ; 366 : 1423 – 1432 .
Chuah S K . Transient ventricular arrhythmia as a cardiac manifestation in dengue haemorrhagic fever – a case report . Singapore Med J 1987 ; 28 : 569 – 572 .
Horta V H , Ferreira Junior J A , Braga de Paiva J M et al. . Acute atrial fibrillation during dengue hemorrhagic fever . Braz J Infect Dis 2003 ; 7 : 418 – 422 .
Wichmann D , Kularatne S , Ehrhardt S et al. . Cardiac involvement in dengue virus infections during the 2004/2005 dengue fever season in Sri Lanka . Southeast Asian J Trop Med Public Health 2009 ; 40 : 727 – 730 .
Salgado D M , Panqueba C A , Castro D et al. . Myocarditis in children affected by dengue hemorrhagic fever in a teaching hospital in Colombia . Rev Salud Publica (Bogota) 2009 ; 11 : 591 – 600 .
Wali J P , Biswas A , Chandra S et al. . Cardiac involvement in dengue haemorrhagic fever . Int J Cardiol 1998 ; 64 : 31 – 36 .
Weerakoon K G , Kularatne S A , Edussuriya D H et al. . Histopathological diagnosis of myocarditis in a dengue outbreak in Sri Lanka, 2009 . BMC Res Notes 2011 ; 4 : 268 .
Donegani E , Briceno J . Disorders of atrio-ventricular conduction in patients with hemorrhagic dengue . Minerva Cardioangiol 1986 ; 34 : 477 – 480 .
Promphan W , Sopontammarak S , Pruekprasert P et al. . Dengue myocarditis . Southeast Asian J Trop Med Public Health 2004 ; 35 : 611 – 613 .
Punja M , Mark D G , McCoy J V et al. . Electrocardiographic manifestations of cardiac infectious-inflammatory disorders . Am J Emerg Med 2010 ; 28 : 364 – 377 .
Cooper L T Jr . Myocarditis . N Engl J Med 2009 ; 360 : 1526 – 1538 .
Salgado D M , Eltit J M , Mansfield K et al. . Heart and skeletal muscle are targets of dengue virus infection . Pediatr Infect Dis J 2010 ; 29 : 238 – 242 .
Araujo S A , Moreira D R , Veloso J M et al. . Fatal Staphylococcal infection following classic Dengue fever . Am J Trop Med Hyg 2010 ; 83 : 679 – 682 .
Kohli U , Sahu J , Lodha R et al. . Invasive nosocomial aspergillosis associated with heart failure and complete heart block following recovery from dengue shock syndrome . Pediatr Crit Care Med 2007 ; 8 : 389 – 391 .
- cardiac myocytes
- myocarditis
- myocarditis, acute
- cardiogenic shock
- dengue fever
- dengue virus
Email alerts
More on this topic, related articles in pubmed, citing articles via.
- Recommend to Your Librarian
- Advertising and Corporate Services
- Journals Career Network
Affiliations
- Online ISSN 2048-8734
- Copyright © 2024 European Society of Cardiology
- About Oxford Academic
- Publish journals with us
- University press partners
- What we publish
- New features
- Open access
- Institutional account management
- Rights and permissions
- Get help with access
- Accessibility
- Advertising
- Media enquiries
- Oxford University Press
- Oxford Languages
- University of Oxford
Oxford University Press is a department of the University of Oxford. It furthers the University's objective of excellence in research, scholarship, and education by publishing worldwide
- Copyright © 2024 Oxford University Press
- Cookie settings
- Cookie policy
- Privacy policy
- Legal notice
This Feature Is Available To Subscribers Only
Sign In or Create an Account
This PDF is available to Subscribers Only
For full access to this pdf, sign in to an existing account, or purchase an annual subscription.
Thank you for visiting nature.com. You are using a browser version with limited support for CSS. To obtain the best experience, we recommend you use a more up to date browser (or turn off compatibility mode in Internet Explorer). In the meantime, to ensure continued support, we are displaying the site without styles and JavaScript.
- View all journals
- My Account Login
- Explore content
- About the journal
- Publish with us
- Sign up for alerts
- Open access
- Published: 25 April 2024
Travel surveillance uncovers dengue virus dynamics and introductions in the Caribbean
- Emma Taylor-Salmon 1 , 2 na1 ,
- Verity Hill 2 na1 ,
- Lauren M. Paul ORCID: orcid.org/0000-0001-5503-7570 3 na1 ,
- Robert T. Koch 2 na1 ,
- Mallery I. Breban 2 ,
- Chrispin Chaguza 2 ,
- Afeez Sodeinde 2 ,
- Joshua L. Warren 4 , 5 ,
- Sylvia Bunch 6 ,
- Natalia Cano 6 ,
- Marshall Cone 6 ,
- Sarah Eysoldt 6 ,
- Alezaundra Garcia 6 ,
- Nicadia Gilles 6 ,
- Andrew Hagy 6 ,
- Lea Heberlein 6 ,
- Rayah Jaber 6 ,
- Elizabeth Kassens 6 ,
- Pamela Colarusso 7 ,
- Amanda Davis 7 ,
- Samantha Baudin 8 ,
- Edhelene Rico 8 ,
- Álvaro Mejía-Echeverri 8 ,
- Blake Scott 9 ,
- Danielle Stanek 9 ,
- Rebecca Zimler ORCID: orcid.org/0009-0006-4991-4016 9 ,
- Jorge L. Muñoz-Jordán 10 ,
- Gilberto A. Santiago 10 ,
- Laura E. Adams 10 ,
- Gabriela Paz-Bailey 10 ,
- Melanie Spillane 11 , 12 ,
- Volha Katebi 11 ,
- Robert Paulino-Ramírez 13 ,
- Sayira Mueses 13 ,
- Armando Peguero 13 ,
- Nelissa Sánchez 13 ,
- Francesca F. Norman ORCID: orcid.org/0000-0003-0336-7767 14 ,
- Juan-Carlos Galán ORCID: orcid.org/0000-0002-8447-0960 15 ,
- Ralph Huits ORCID: orcid.org/0000-0001-8803-9468 16 ,
- Davidson H. Hamer 17 ,
- Chantal B. F. Vogels ORCID: orcid.org/0000-0003-0027-6480 2 , 18 na2 ,
- Andrea Morrison ORCID: orcid.org/0009-0006-8686-2841 9 na2 ,
- Scott F. Michael ORCID: orcid.org/0000-0003-3236-2017 3 na2 &
- Nathan D. Grubaugh ORCID: orcid.org/0000-0003-2031-1933 2 , 5 , 18 , 19 na2
Nature Communications volume 15 , Article number: 3508 ( 2024 ) Cite this article
714 Accesses
22 Altmetric
Metrics details
- Computational models
- Dengue virus
- Epidemiology
- Molecular evolution
Dengue is the most prevalent mosquito-borne viral disease in humans, and cases are continuing to rise globally. In particular, islands in the Caribbean have experienced more frequent outbreaks, and all four dengue virus (DENV) serotypes have been reported in the region, leading to hyperendemicity and increased rates of severe disease. However, there is significant variability regarding virus surveillance and reporting between islands, making it difficult to obtain an accurate understanding of the epidemiological patterns in the Caribbean. To investigate this, we used travel surveillance and genomic epidemiology to reconstruct outbreak dynamics, DENV serotype turnover, and patterns of spread within the region from 2009-2022. We uncovered two recent DENV-3 introductions from Asia, one of which resulted in a large outbreak in Cuba, which was previously under-reported. We also show that while outbreaks can be synchronized between islands, they are often caused by different serotypes. Our study highlights the importance of surveillance of infected travelers to provide a snapshot of local introductions and transmission in areas with limited local surveillance and suggests that the recent DENV-3 introductions may pose a major public health threat in the region.
Similar content being viewed by others
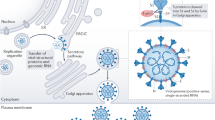
Mechanisms of SARS-CoV-2 entry into cells
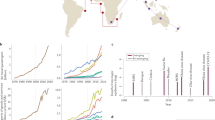
Infectious disease in an era of global change
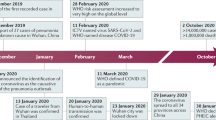
Characteristics of SARS-CoV-2 and COVID-19
Introduction.
Dengue is an acute febrile illness viral infection caused by dengue virus (DENV), which is transmitted to humans through the bite of an infected Aedes mosquito 1 , 2 , 3 . There are four genetically- and antigenically-related DENV serotypes, and while infection with one serotype generally produces long-lasting homotypic immunity, it only induces short-lived heterotypic immunity, after which infection with another serotype may result in more severe disease 1 , 2 , 4 . Almost half of the world’s population lives in dengue-endemic areas, with children bearing the majority of the disease burden 5 , 6 . Dengue has evolved from a sporadic disease into a major public health threat in the Americas, with reported case numbers reaching 20 million in the last 5 years in 46 countries 7 . The Caribbean has often been a source of reintroduction and spread of all four DENV serotypes over the last thirty years 8 , 9 . Two other Aedes -borne viruses also emerged in this region: chikungunya virus in 2013 10 , 11 and Zika virus in 2016 12 , 13 . Local infectious disease surveillance and reporting is essential for public health efforts regarding outbreak response and disease mitigation; however, the control of outbreaks is challenging in low- and middle-income countries where resources are limited 14 . In addition, dengue outbreaks can overwhelm health systems, further weakening surveillance capacity - particularly in countries that may not have strong laboratory infrastructure 15 , 16 , 17 . Therefore, the areas where disease surveillance is the most needed - like the Caribbean - are often the same areas where we have limited publicly available data.
Infectious disease surveillance of travelers has been shown to supplement local surveillance in low-resource areas 18 , 19 , 20 , 21 , 22 , 23 , 24 . Infected travelers can also be sentinels for pathogen transmission in locations where outbreaks have not yet been reported 25 . For example, characterization of pathogens in infected travelers was used to reconstruct the global spread of H1N1 in 2009 26 and an unreported Zika outbreak in Cuba in 2017 18 . Utilizing traveler data could be particularly useful for supplementing surveillance in the Caribbean because of the popularity of tourism in the region 27 . In the United States, dengue is the leading cause of febrile illness among travelers returning from the Caribbean 23 , 25 , 28 , 29 , 30 . In Florida, the number of travel-associated dengue cases has increased dramatically in recent years 31 . Florida is a good sentinel for the Caribbean, due to its geographic location and high volume of travel back and forth between Florida and the islands. Therefore, we hypothesize that we can use surveillance of dengue-infected travelers diagnosed in Florida who recently returned from the Caribbean to better reconstruct DENV dynamics in the region.
In this study, we combined surveillance and sequencing of DENV from infected travelers to estimate local outbreak sizes, track serotypes, and map the patterns of lineage spread from 2009 to 2022. We determined that travel-associated infections closely mirrored local rates for countries and territories with robust local surveillance. An increase in infected travelers returning from Cuba in 2022 has previously been reported 32 , leading to the assumption of a large dengue outbreak that was not reported to Pan-American Health Organization (PAHO), the primary source for information regarding dengue spread in the Americas 7 . We used surveillance data among travelers to estimate that the 2022 outbreak in Cuba was similar in size to other large outbreaks reported in the Americas. We used serotype data to decipher inter-island differences during outbreak years. By sequencing DENV isolates from travel associated cases, we uncovered two recent DENV-3 introductions from Asia into Jamaica and Cuba, the latter of which has now been detected in several other locations in the region 33 . Overall, our study highlights the importance of dengue surveillance among travelers and genomic epidemiology in supplementing local infectious disease surveillance in resource-limited locations by elucidating DENV transmission and spread within the Caribbean.
Dengue travel cases to supplement local surveillance
Dengue incidence in the Americas rose significantly over the last four decades, reemerging as a major public health concern and culminating in ~17.5 million reported cases from 2010 to 2019 and ~20 million cases since 2019 7 . This coincided with a rapid increase in travel-associated dengue cases within the United States, especially in Florida. The Florida Department of Health (FDOH) has a robust dengue surveillance system, which captures symptomatic cases reported in patients who traveled to a dengue-endemic area within the two weeks prior to illness onset (Table S1 ). From 2009 to 2022, the FDOH reported between 19 (in 2017) to 929 (in 2022) travel-associated dengue cases per year, for a total of 2300 cases, with the majority (1815 cases, 78.9%) occurring in travelers who recently returned from the Caribbean (Supplementary Fig. S1 ). Overall, four countries and one US territory made up 75.6% (1737 cases) of all travel-associated dengue cases reported in Florida from 2009 to 2022: Cuba, Puerto Rico, Dominican Republic, Haiti, and Jamaica (Fig. 1 ). There is significant variability in local dengue surveillance and reporting among Caribbean countries and territories, which can impact local outbreak responses and travel advisories 34 , 35 . We hypothesize that we can address this variability by using dengue surveillance of infected travelers in Florida to detect surveillance gaps within these five Caribbean islands.
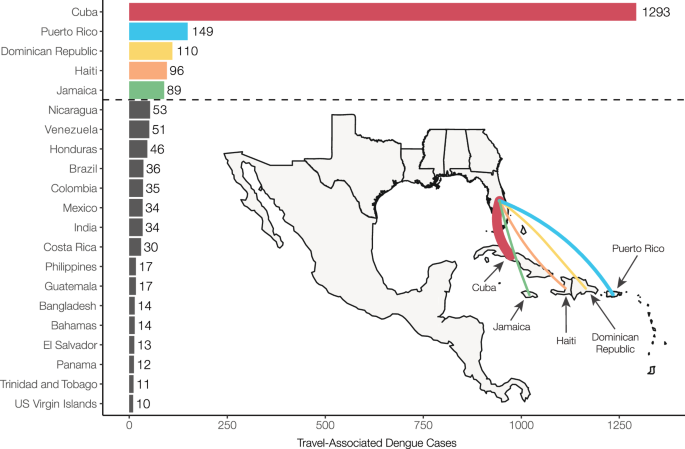
Countries and territories are listed by total number of travel cases for each inferred origin of infection based on travel history, in descending order. Only countries or territories with at least 10 associated travel infections are shown. The complete data can be found in Supplementary Table S1 . The inset shows a map of the location of the top 5 associated country origins of travel cases reported in Florida, with the line width proportional to the number of travel cases.
To determine the extent to which travel-associated cases reported in Florida correlated with local reporting in the Caribbean, we compared local and travel-associated dengue case numbers from 2009 to 2022 (Fig. 2 ). We obtained yearly suspected and confirmed dengue cases reported by Cuba, Dominican Republic, Haiti, Jamaica, and Puerto Rico from PAHO and all reports of travel-associated dengue cases from the FDOH. First, we compared the local and travel case trends for each country or territory. We found significant positive correlations from the Dominican Republic (Pearson r = 0.764, p = 0.001), Jamaica ( r = 0.960, p < 0.001), and Puerto Rico ( r = 0.911, p < 0.001), but the data from Cuba ( r = 0.496, p = 0.070) and Haiti ( r = −0.694, p = 0.056) were not correlated (Fig. 2A ). We then used flight data from the United States Department of Transportation (Supplementary Fig. S2 ) to estimate the ‘travel infection rates’ as the number of travel-associated cases per 100,000 air passenger journeys into Florida per year from each location 18 , 36 . Comparing the travel infection rates to the local rates (dengue cases per 100,000 population), we again found positive correlations from the Dominican Republic (Pearson r = 0.750, p = 0.002), Jamaica ( r = 0.912, p < 0.001), and Puerto Rico ( r = 0.935, p < 0.001), but not from Cuba ( r = 0.458, p = 0.1) and Haiti ( r = −0.825, p = 0.012; Fig. 2B ). The strong correlations between travel and local cases from the Dominican Republic, Jamaica, and Puerto Rico are similar to what we previously estimated for Zika 18 and others estimated for dengue 36 , reflecting the robust and consistent arbovirus surveillance systems at these locations. Notably, we found peaks of travel-associated infections from Cuba in 2022 that were not captured by the dengue case data reported by PAHO (Fig. 2A ). This increase in travel-associated cases was also seen in other parts of the world 32 . Our data also show disagreements between travel and local dengue cases in Haiti, specifically spikes in travel-associated cases in 2012 and 2018 and a spike in local cases in 2021 that were not reflected in the other data (Fig. 2A ). In fact, there are many years of missing dengue case data in the PAHO repository from Haiti, and these gaps are frequently paired with natural disasters (e.g. 2010 earthquake). Overall, we demonstrate here that dengue travel surveillance in Florida can help supplement local surveillance in the Caribbean region to infer relative local case dynamics.
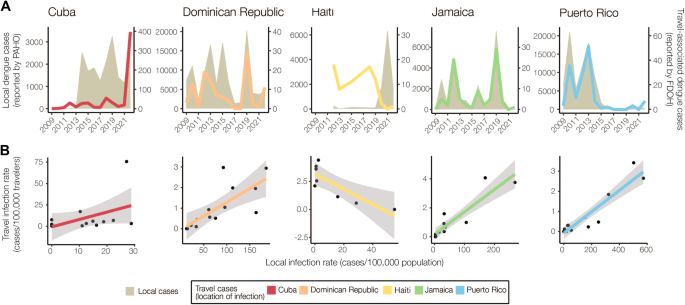
A Yearly local dengue cases (left y-axis, gray shaded area) reported by PAHO and yearly travel-associated dengue cases (right y-axis, colored lines) reported by FDOH were sorted by the origin of exposure. The datasets were compared using Pearson’s correlation coefficient. There were strong positive correlations between travel and local cases for the Dominican Republic (Pearson r = 0.764, p = 0.001), Jamaica ( r = 0.960, p < 0.001), and Puerto Rico ( r = 0.911, p < 0.001), with no significant correlation for Cuba ( r = 0.498, p = 0.070) and a negative correlation for Haiti ( r = −0.694, p = 0.056). B The local dengue virus incidence rates for each country or territory were calculated by the number of locally reported cases per month per 100,000 population. The travel dengue virus incidence rates for each country or territory of presumed exposure were calculated by the number of travel-associated cases per month per 100,000 air passenger journeys entering Florida from endemic locations. Colored lines represent a two-sided linear regression model with local infection rate as the predictor variable and travel infection rate as the outcome variable. Gray shaded area represents standard error. There were strong positive correlations between travel and local incidence for the Dominican Republic (Pearson r = 0.750, p = 0.002), Jamaica ( r = 0.912, p < 0.001), and Puerto Rico ( r = 0.935, p < 0.001), with no significant correlation for Cuba ( r = 0.458, p = 0.100) and a negative correlation for Haiti ( r = −0.825, p = 0.012). The negative correlation between the local and travel infection rates may have been driven by a decreased travel volume to Florida from 2020 to 2022 (Supplementary Fig. S2 ).
Estimating local dengue infection rates using travelers
Having demonstrated that the Dominican Republic, Jamaica, and Puerto Rico have significant positive correlations between local and travel-associated dengue infection rates (travel cases per 100,000 air passenger journeys; Fig. 2 ), we then created a negative binomial regression model using these relationships to estimate local infection rates from Cuba and Haiti. We estimate that the 2022 dengue outbreak in Cuba is larger than any detected on the island since at least 2010 and similar in size to other large outbreaks found throughout the Americas (Fig. 3 ).
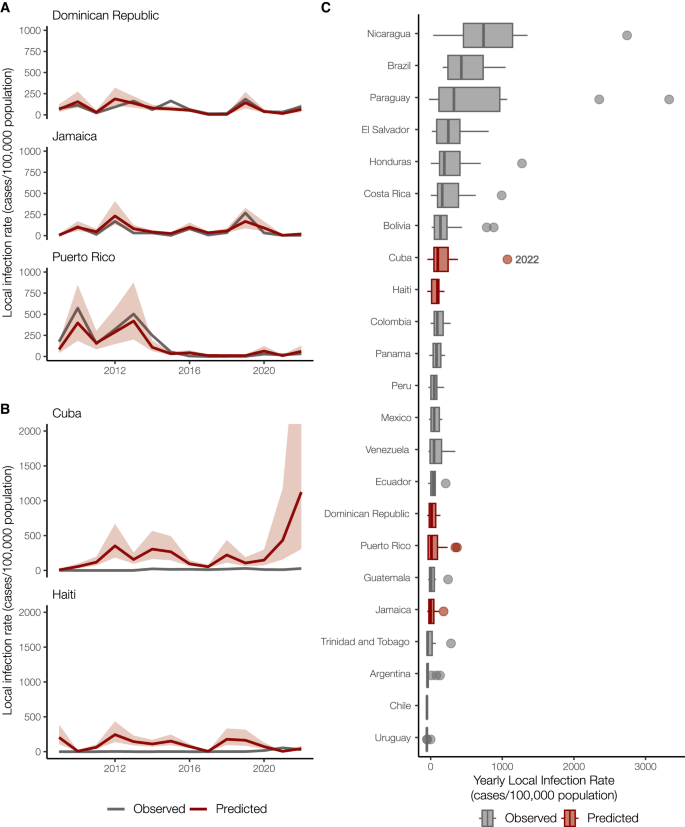
A Local and travel-associated dengue infection rates from the Dominican Republic, Jamaica, and Puerto Rico were used as predictors in a negative binomial regression model due to the strong correlations between local and travel-associated cases. The red lines indicate the predicted mean infection rates, and the shaded area indicates the 95% confidence interval, while the gray lines indicate the local dengue cases as reported to PAHO. B Local dengue infection rates in Cuba and Haiti from 2009 to 2022 were predicted using the model from panel A . The colored lines indicate the predicted mean infection rates, and the shaded area indicates the 95% prediction interval, while the gray lines indicate the local dengue cases as reported to PAHO. C Observed and predicted local infection rates for South American and Caribbean countries and territories with populations over one million inhabitants from 2009 to 2023. Within each box, thick vertical lines denote median values (50 th percentile); boxes extend from the 25 th to the 75 th percentile; horizontal lines extending from boxes denote mark the 5 th and 95 th percentiles, and the dots denote outliers, representing large outbreaks from any year. Locations in gray with observed cases were not included in our model.
In areas with inadequate local case reporting, previous studies suggested that sentinel surveillance of infected travelers can be used to estimate virus transmission dynamics and spread from endemic locations 18 , 26 , 36 . Only 3036 laboratory-confirmed dengue cases were reported by Cuba in 2022, similar to what was reported in 2019 7 . This is inconsistent with the number of cases we detected from Cuba using travel surveillance (Figs. 1 and 2A ). Moreover, dengue case data from Haiti were not reported to PAHO for 2009–2011, 2014, and 2016–2017 7 . To estimate the number of cases that likely went under-reported in Cuba and Haiti during our study period, we constructed a negative binomial regression model to predict local infection rates from the travel rates (Fig. 3A ). We found that when controlling for the gross domestic product (GDP; a relative approximation of resources available for surveillance), population, and year, the risk associated with local cases of dengue increases by 96% (95% confidence interval [CI]: 63–134%) for every one unit increase in the log of travel infection rates from the Dominican Republic, Jamaica, and Puerto Rico.
When applying our model to travel data from Cuba and Haiti, we estimate new dengue case dynamics (Fig. 3B ) and compare the predicted infection rates to other observed rates throughout the Americas (Fig. 3C ). We estimate that the average yearly dengue infection rates from Haiti (244 [95% CI: 138–434]/100,000 population) are similar to the reported and estimated rates from the Dominican Republic (188 [95% CI: 111–320]/100,000), which is reasonable given that they are on the same island. We also estimate that from 2010-2021, the peaks in dengue infection rates from Cuba were similar in magnitude to other Caribbean islands (~500/100,000 population). However, we predict that Cuba likely experienced a large outbreak in 2022 (~1000 [95% CI: 306–4117]/100,000 population), potentially one of the largest in the region. Our reconstructed dengue infection rates using travel surveillance provide a clearer picture of dynamics in the Caribbean and allow us to investigate previously unreported trends.
Asynchrony of dengue virus serotypes across locations
Using travel-associated dengue cases, we reconstructed the infection trends in different locations of the Caribbean (Fig. 3 ). To obtain a deeper resolution of the outbreaks, we determined the DENV serotype from infected individuals with recent travel to the Caribbean and estimated the annual proportions from each location from 2010 to 2022. We discovered that outbreaks on different islands were often caused by different serotypes, even when those outbreaks occurred during the same years (Fig. 4 ).
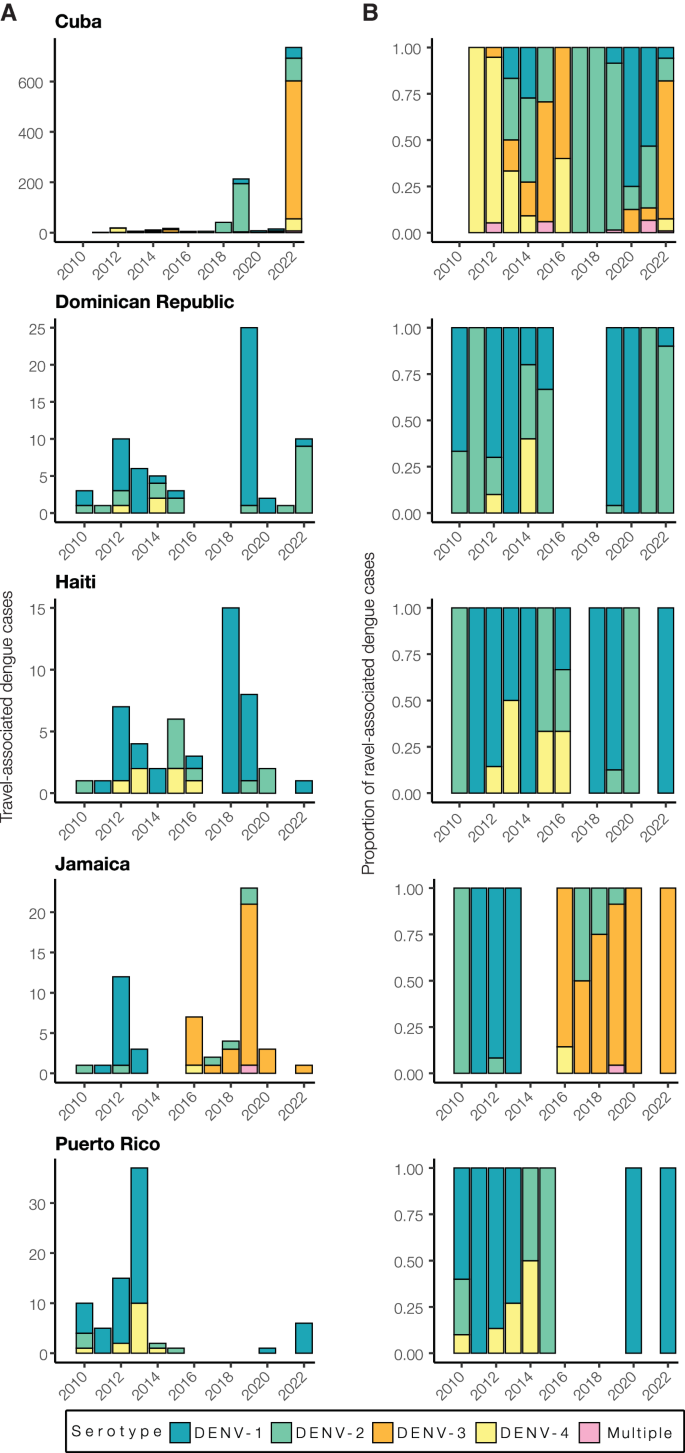
A Yearly travel-associated dengue cases by serotype were reported by FDOH and sorted by country or territory of likely exposure. B The relative proportions of yearly travel-associated dengue cases by serotype per year and per country or territory of likely exposure, normalized to account for the number of infected travelers.
While some countries report DENV serotype data associated with cases to PAHO, the data are released to the public as a binomial (present or absent) without accompanying proportions. Therefore, it is often difficult to determine (1) which serotypes are mostly responsible for outbreaks and (2) the temporal trends that may be useful for forecasting. To overcome this data limitation, we determined the serotype data for all travel-associated dengue cases from the FDOH from 2010 to 2022 (2009 serotype data were not available; Fig. 4 ; Supplementary Table S1 ). We analyzed the data as the total number of travel cases by serotype (Fig. 4A ) and the proportion of each serotype (Fig. 4B ) per year and country or territory. As a form of validation, our serotype proportions estimated from infected travelers returning from Puerto Rico generally match the proportions from local cases reported from Puerto Rico, including the dominance of DENV-1 from 2010 to 2013, the rise in DENV-4 from 2012 to 2014, the rise of DENV-2 from 2014 to 2015, and the re-emergence of DENV-1 in 2020 37 . Overall, we found multiple serotypes for several years and locations, which has often been reported, but years with high travel infection rates were typically dominated by a single serotype (e.g. DENV-3 from Cuba in 2022 and DENV-1 from the Dominican Republic in 2019).
As is expected for DENV, we found that different serotypes transitioned in and out of dominance in each location during the twelve years (Fig. 4 ). This is consistent with genotype replacement events, which occur when a previously dominant lineage is replaced by another related, but distinct, lineage 38 , 39 , 40 . Various theories have been proposed to explain these events, including natural selection, immune pressure, and population bottlenecks. During the earlier years, however, we did detect some patterns. We found that from 2010 to 2016, DENV-1 and DENV-2 predominated in the Dominican Republic, Haiti, Jamaica, and Puerto Rico. Immediately following the 2015-2016 Zika epidemic, dengue cases decreased throughout the Americas 41 . As dengue cases began to increase again to record highs in 2019 (>3 million cases reported to PAHO), we found that many outbreaks were caused by different serotypes. The most prevalent serotypes in 2019 were DENV-1 from the Dominican Republic (96%, n = 25) and Haiti (88%, n = 8), DENV-2 from Cuba (90%, n = 213), and DENV-3 from Jamaica (87%, n = 23). By 2022, DENV-1 was the most commonly detected serotype in Puerto Rico (100%, n = 6), DENV-2 arose in the Dominican Republic (90%, n = 10), and DENV-3 became the new dominant serotype in Cuba (74%, n = 735). The only location that consistently did not follow other Caribbean serotype trends, even before the Zika epidemic, was Cuba. Overall, we demonstrate that dengue outbreaks in the Caribbean cannot be treated as a single entity, even when they are synchronized throughout the region (e.g. 2019), as transmission in different islands can be due to different DENV serotypes regardless of temporal relationships.
Sequencing travel infections reveals dengue virus diversity in the Caribbean
Our analyses show that dengue outbreaks in the Caribbean exhibit serotype variability between islands despite temporal synchrony (Fig. 4 ). While serotype-level data can provide broad-scale information, there is substantial within-serotype diversity. Therefore, to uncover the DENV genetic diversity and patterns of spread within the Caribbean, we sequenced 295 traveler infections (Fig. 5 ).
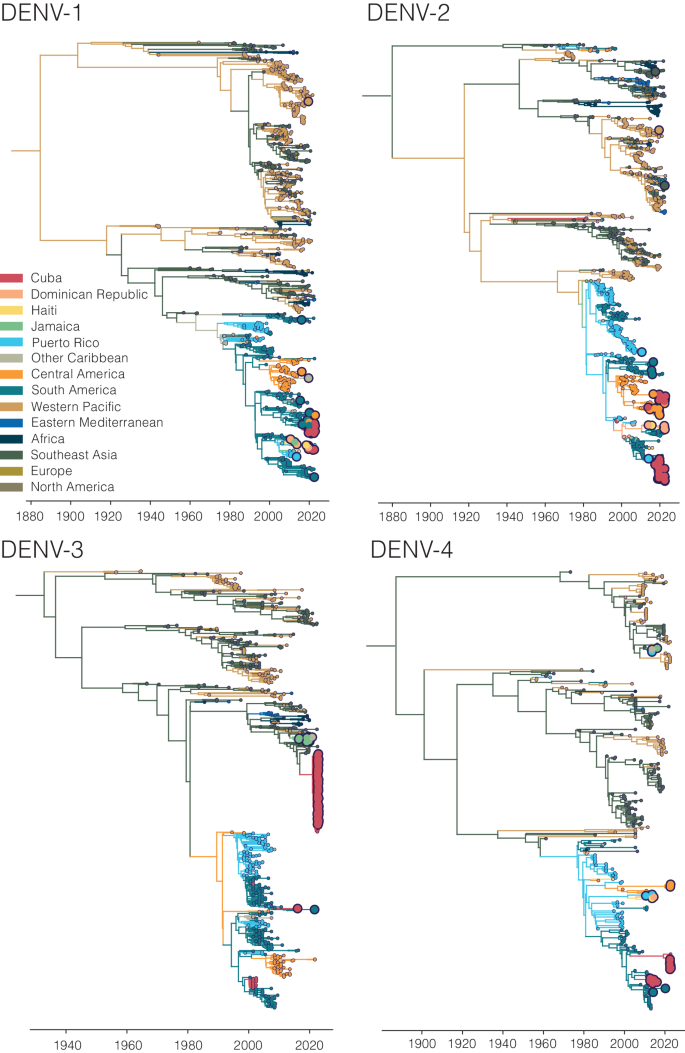
Time-resolved phylogenies of each serotype, with the branches and tips colored by inferred and sampled locations respectively. Larger dots represent those DENV samples sequenced for this study.
Using sequencing data that we generated from travel-associated dengue cases, we performed four discrete phylogeographic analyses, one for each serotype (Fig. 5 ). The travel origin location in our analysis was designated as the site of presumed DENV exposure. We subdivided the Caribbean into Cuba, Dominican Republic, Haiti, Jamaica, Puerto Rico, and “other Caribbean”. For context, we used genomic data from North America, South America, and Central America, and representative DENV sequences sorted by WHO regions for the other areas of the world to highlight viral transmission into and within the Caribbean. Our data revealed the continued circulation of all four DENV serotypes and multiple clades within those serotypes in different parts of the Caribbean as recently as 2022. While most of the sequenced travel infections cluster within clades that have historically circulated within their respective regions, we also detected several introductions of all four serotypes into the Caribbean from southeast Asia, the western Pacific, and elsewhere from the Americas stretching back to the 1970s to as recently as 2021 (described in more detail in the next section; Fig. 6 ).
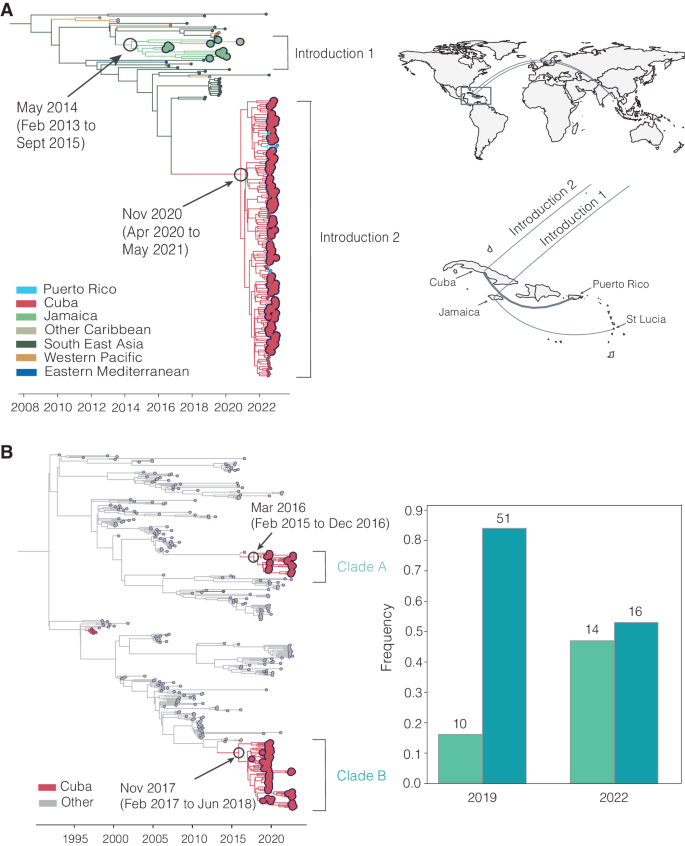
A Time-revolved phylogeny showing global spread of DENV-3 genotype III. The times of the most common ancestor of the clades are taken conservatively as introduction times, with 95% HPDs indicated in parentheses, and are indicated by circles. The map shows the transitions from Southeast Asia to Cuba and Jamaica and within the Caribbean. Countries or territories involved are indicated on the map, and virus transitions are indicated by lines going counter-clockwise, with thickness indicating a number of movements. B Time-resolved phylogeny shows two co-circulating DENV-2 (genotype III) clades in Cuba, which are colored in red, with 95% HPDs indicated in parentheses. The bar chart shows the frequencies of each clade in 2019 and 2022.
To support our phylogenetic analyses, we performed three independent validations. One of our primary findings is the detection of a large DENV-3 (genotype III) clade from infected travelers returning from Cuba (Figs. 5 and 6A ). First, we sequenced two samples from DENV-infected travelers returning from Cuba to Spain in 2022 and, second, we obtained sequencing data generated by the CDC Dengue Branch from travelers infected in Cuba in 2022, also provided by the FDOH. All of these sequences clustered in the same clade with the rest of the sequences from Cuba travelers. Third, we also sequenced samples from local dengue cases from the Dominican Republic collected in 2022 and found that these DENV-2 sequences clustered with DENV-2 sequences from Dominican Republic travel-associated dengue cases. These data suggest that our DENV genetic clustering patterns are not unique to our study population and sequencing methods, and likely represent local diversity.
Phylogenetic patterns of emergence, spread, and transmission
Our analyses show that infected travelers can reveal DENV diversity within the Caribbean (Fig. 5 ). We next used our genomic data to uncover patterns of DENV introductions, spread, and transmission in the region (Fig. 6 ). Our intent here is not to explore every location-specific detail, but to showcase what is possible with even a relatively limited number of sequenced DENV genomes from travelers.
In our analysis, we detected a substantial number of travel-associated dengue cases coming from Cuba during 2022 (Fig. 2 ), and we estimated that these stemmed from a large, under-reported local outbreak (Fig. 3 ) predominantly caused by DENV-3, genotype III (Figs. 4 and 5 ). Our phylogeographic analysis further shows that the large cluster of DENV-3 sequences from Cuba in 2022 (148 Cuban sequences out of 150 total sequences in the clade, posterior support for location = 1.0) was from an introduction directly or indirectly from southeast Asia that occurred by at least late 2020 (95% HPD = 2020-04-06 to 2021-05-13; Fig. 6A ). We and others found that this lineage has already spread to Puerto Rico, Florida, Arizona, and Brazil by 2022 33 , 42 . Thus, this may be a rapidly spreading lineage of concern for the Americas.
We discovered that the 2022 Cuba outbreak clade was not the first recent reintroduction of DENV-3, genotype III, into the Caribbean from southeast Asia. Sequencing travel-associated infections also revealed an earlier but distinct introduction of this genotype into Jamaica (all 11 sequences in the clade, posterior support for location = 1.0) by at least 2014 (95% HPD = 2013-02-02 to 2015-09-04; Fig. 6A ). We first detected this lineage from three travelers returning from Jamaica in 2016 and then again from 7 travelers during the re-emergence of DENV-3 in Jamaica in 2019 (Fig. 4 ). We last sequenced this lineage from a traveler from St. Lucia in late 2020, indicating that the virus had limited spread, but likely not as extensively as the Cuba lineage.
In addition to lineage introductions, we also explored within-location lineage dynamics. In particular, we detected instances of multiple lineages from the same serotype co-circulating in the same country. For example, we detected two co-circulating lineages of DENV-2 (genotype III) in Cuba, designated here as clades A and B (Fig. 6B ). We estimate that they were introduced into Cuba by at least 2016 (95% HPD 2015-12-20 to 2016-12-15, likely from Puerto Rico, posterior support = 0.77) and late 2017 (95% HPD 2017-02-20 to 2018-06-25, likely from Central America or Mexico, posterior support = 1.0), respectively, and both continued to circulate through the 2019 and 2022 outbreaks. Their relative frequencies changed between 2019 and 2022, with clade B dominating in 2019 but clade A increasing to become more even in frequency in 2022, though these trends may be impacted by sampling biases. We also found evidence of co-circulating DENV-1 clades in Cuba (Fig. 5 ). While neither of these clades has any concerning mutational signals, evaluating the transmissibility of co-circulating lineages is the basis of discovering meaningful phenotypes that may impact the effectiveness of interventions.
Travel surveillance and genomic epidemiology uncover dengue virus dynamics
Using patterns of infected travelers and virus genomics, we discovered previously unrecognized DENV dynamics within the Caribbean. Dengue is a major public health concern within the Caribbean, and the past two decades have seen significant increases in morbidity and mortality in the region 5 , 34 , 43 . With dengue outbreaks increasing in frequency and severity 43 , accurate and detailed surveillance data are crucial for epidemiological investigations. Currently, the main source of information about dengue outbreaks in the Caribbean comes from PAHO and other public health organizations, which rely on accurate case reporting from national or territorial health agencies. However, previous studies have shown large differences between case reporting to PAHO and estimated cases 34 .
Dengue surveillance among travelers can be an effective method to supplement local surveillance, especially in resource-limited settings 18 , 19 , 20 , 32 . There is a large volume of travel between Florida and the Caribbean (Supplementary Fig. S2 ), and Florida has experienced a steady increase in imported dengue cases over the last two decades, especially from the Caribbean (Fig. 1 ) 44 . In this study, we investigated whether travelers with dengue returning to Florida could be used as a surrogate for local transmission in the Caribbean and found significant positive correlations between local and travel infection rates for the Dominican Republic, Jamaica, and Puerto Rico (Fig. 2B ). For locations with local surveillance gaps, we built a model to estimate local infection rates in these areas, assuming the similarity between local and travel data (Fig. 3 ). From this analysis, we uncovered a large dengue outbreak in Cuba in 2022 (Fig. 3B ) and estimated that it was similar in size to other large outbreaks detected elsewhere in the Americas (Fig. 3C ). To investigate the DENV serotypes responsible for these outbreaks, we analyzed serotype data from infected travelers returning to Florida and showed that these outbreaks are not synchronized across islands, with different serotypes responsible for outbreaks on different islands within the same year (Fig. 4 ). We then used viral genomics to provide a snapshot of genetic diversity within the region (Fig. 5 ) and uncover DENV spread and introductions (Fig. 6 ). Overall, we demonstrate that using information about dengue in travelers, including the use of sequencing, should become more routinely used to enhance dengue surveillance.
New DENV-3 introductions from Asia led to a large outbreak in Cuba
There have been several reintroductions of all four DENV serotypes into the Americas since its reemergence during the 1960–80s. Here, we detected two recent DENV-3 (genotype III) introductions into Cuba and Jamaica. We estimate that the introduction from southeast Asia into Cuba occurred by late 2020 (Fig. 6A ) and, in the course of only two years, caused a large outbreak (Fig. 3B ) and spread to several other locations in the Americas 33 . Given the speed of these events, it is possible that this DENV-3 lineage has a transmission advantage over at least some DENV lineages in the Americas.
This is not the first time that DENV-3 re-emerged in the Americas leading to widespread outbreaks. Historically, DENV-3 was re-introduced in the Americas in the 1970s, but then was not detected for 16 years between 1978 and 1994, when it was almost simultaneously isolated from Nicaragua and Panama 45 , 46 , 47 . It subsequently spread throughout Central America and the Caribbean, leading to widespread epidemics of dengue hemorrhagic fever 45 , 47 , 48 , 49 . DENV immune waning has previously been observed 4 , 50 , 51 , 52 , 53 , 54 and the sudden DENV-3 re-emergence stemming from that introduction could be attributed to the loss of immunity during the 16-year hiatus, which could be similarly true for the recent DENV-3 introduction into Cuba. Outside of the two new DENV-3 introductions we detected and tracked with travelers, there was very little detection of DENV-3 in the Caribbean (Fig. 3 ) and elsewhere in the Americas over the past 10 years 33 , 55 , 56 . While we do not yet know the true epidemiological impact of this newly introduced lineage, this will be important to closely monitor through local and travel surveillance.
Interestingly, the DENV-3 introduction into Jamaica has not spread to the same extent as the introduction into Cuba, despite its introduction almost 6 years earlier (Fig. 6A ). It could indicate that there are notable transmissibility differences between the two lineages or that the Jamaican lineage did not become established within a population with sufficient susceptibility or mobility to facilitate its spread. While these deterministic and stochastic processes require further investigation, even knowing that this introduction occurred was only possible through our surveillance among travelers - this clade was entirely sequenced from travelers (Fig. 6A ).
Genetic diversity within the region
By sequencing DENV genomes from infected Caribbean travelers, we demonstrated widespread diversity of circulating viral lineages, both between and within serotypes (Figs. 4 and 5 ). Even in outbreak years, such as 2019 or 2022, there was not one lineage that became dominant in the region; instead, different serotypes were often responsible for outbreaks on different islands, even if they occurred at the same time (Fig. 4 ). This indicates synchronized outbreaks are more likely driven by population (e.g. time since last outbreak) and environmental effects (e.g. El Niño years) than virus-related factors.
Most dengue-endemic areas in the Americas have co-circulation of multiple serotypes, which increases the likelihood of concurrent infections 57 , 58 , 59 . In fact, the first-ever confirmed case of concurrent DENV infection was reported in Puerto Rico during the 1982 outbreak in a patient infected with DENV-1 and -4 serotypes 58 , 60 . However, we noted minimal numbers of mixed infections when reviewing serotype data (14 out of 1328, Fig. 4 ), which is significantly lower than those previously reported 57 . Given the size of our dataset, it is possible that the overall likelihood of concurrent infections, even in hyperendemic locations, is actually quite small, as is their overall impact on severe dengue cases and epidemics.
Limitations
Travel surveillance data is limited by location-specific patterns and volumes of travel 19 , 25 . During our study period, there were years in which we detected minimal or no infected travelers despite local dengue cases being reported to PAHO (Fig. 2A ). This introduced biases in our local case estimates (Fig. 3 ) and in our patterns of DENV emergence and spread throughout the region (Fig. 6 ), as we have no travel data from that time period.
For this study, we assumed that the risk of DENV infection was similar for travelers and local inhabitants in endemic countries and territories, meaning that a rise in local cases would correspond to a relative increase in travel-associated infections. However, this may not be the case and would depend on the travelers’ previous exposure to dengue and related flavivirus infections or vaccinations, as well as on the general patterns of travelers’ behaviors while visiting the country or territories (e.g. DENV infections are more common among individuals visiting their friends and relatives than people traveling for other reasons). Endemic locations also experience heterogeneity with regard to dengue risk, as the primary vector for dengue in the Caribbean is Aedes aegypti , which is found more commonly in urban areas. It is also possible that some residents in endemic locations would be less likely to seek medical care and thus be diagnosed with only mild dengue symptoms, as the illness is a common occurrence for them. Thus, using travelers as sentinels alone cannot provide a complete picture of local dengue outbreaks.
Calculating travel infection rates from Cuba has important limitations we tried to address during our analysis. First, due to the associations between local dengue outbreaks in Florida and infected travelers from Cuba, the FDOH enhanced its case finding among individuals reporting recent travel from this location. The enhanced case finding was particularly apparent in 2019 and 2022, when the FDOH reported 413 and 929 travel-associated dengue cases from Cuba, respectively. We attempted to correct this by only using cases in our calculations that would have been detected in those years using the standard practice (i.e. not using “travel to Cuba” as the reason for DENV testing since that is not common practice for travel from other locations). In addition, there was also difficulty with ascertaining an accurate number of travelers from Cuba to Florida, as not all travel between these two areas is documented, including boat travel. We do not expect this to significantly impact our travel volume estimates as we previously thoroughly investigated travel patterns from Cuba to Florida 18 , and undocumented boat travel is estimated to be only 1.18% (6,182/524,611 in 2022) of the travel volumes (air passenger journeys) that we obtained from the US Department of Transportation 61 . The COVID-19 pandemic also impacted travel throughout the region (Supplementary Fig. S2 ) and could have impacted how people interacted with the medical system (e.g. avoiding hospitals, fevers presumed secondary to SARS-CoV-2). This makes it difficult to interpret data from 2020, 2021, and, to some extent, 2022, although for that last year, travel restrictions were mostly lifted, and we detected increased travel within the region (Supplementary Fig. S2 ). Thus, the potential inaccuracies in our travel infection rates from Cuba mean that our modeled local estimates should be viewed as relative rates rather than as absolute values. The compilation of our analysis still indicates that there was a large under-reported dengue outbreak in Cuba in 2022, a finding that was independently discovered from travelers returning from Cuba to other locations 32 .
Utilizing infected travelers as a means of detecting local outbreaks should not replace local dengue surveillance; however, it can be helpful for locations where local surveillance is not feasible or is underfunded. By supplementing local data, travel surveillance can assist with detecting local outbreaks and estimating their size. When combined with genomic epidemiology, we used travel surveillance to provide a snapshot of the diversity of DENV within the Caribbean, as well as reveal patterns of DENV emergence and lineage spread. While we did not address it in this study, travel surveillance can also provide important epidemiological information at the destination (Florida, in this case) and could be incorporated into forecasting models. If this framework was incorporated into a routine system, utilizing networks such as GeoSentinel, over time, it would help support global surveillance efforts to combat the surge of dengue.
The Institutional Review Boards (IRB) from the FDOH, Universidad Iberoamerican (UNIBE), Florida Gulf Coast University, and the Yale University Human Research Protection Program determined that pathogen genomic sequencing of de-identified remnant diagnostic samples as conducted in this study is not research involving human subjects (Yale IRB Protocol ID: 2000033281; UNIBE-CEI# 2021-88).
Travel-associated cases, serotypes, and infection rates
Weekly cumulative reports on travel-associated dengue case numbers were collected from 2009 to 2022 and are publicly available from the FDOH 62 . These cases reported on the FDOH database include those that were confirmed by both PCR and serologic assays. Serotype data for travel-associated dengue cases was determined by the FDOH using the CDC DENV-1–4 RT-PCR Assay 63 . Travel-associated cases occurred in individuals who had traveled to a dengue-endemic country or territory in the two weeks prior to symptom onset. For this study, we only included patients who traveled to one endemic location within the 2 weeks prior to symptoms onset so we could more accurately sort the temporal and spatial distribution of travel-associated cases. This led to the exclusion of 28 patients who traveled to multiple countries during our study period, constituting 1.2% of the 2300 total cases (Supplementary Table S1 ). Within the Caribbean, the focus of this paper, there were 18 patients that were excluded due to traveling to multiple countries, constituting 1% of the 1815 total cases. Therefore, due to the small number of these cases, we did not feel that excluding them would affect our analysis. We aggregated the data by year and by location of likely exposure (i.e., travel origin).
Dengue is a reportable disease in the United States. In addition to health care provider and laboratory reporting, the FDOH uses syndromic surveillance to aid in case identification. FDOH detected syndromic surveillance cases by searching de-identified chief complaints, admission and discharge diagnoses, travel-related fields, etc., obtained from participating emergency rooms and urgent care centers in Florida. Information from suspected dengue cases are forwarded to the county health departments, who in turn reach out to the local hospitals and ascertain whether dengue testing was ordered. Enhanced surveillance was increased during 2019 and 2022 due to the large number of travel-associated cases being detected. These efforts involved adding recent travel to Cuba as a criterion, in addition to dengue-like symptoms. Eighteen of the 413 travel-associated cases in 2019 and 397 of the 929 cases in 2022 were first identified via syndromic surveillance and used in our analysis. Of note, 116 cases identified via enhanced surveillance in 2022 would not have met our case definition without this additional criterion. Therefore, in order to counter these differences in case ascertainment, we only included cases captured via traditional reporting, not enhanced detection of cases among travelers returning from Cuba, for our calculations.
When we compared the local and travel case trends for Cuba with versus without the enhanced surveillance, we found that excluding these travel cases led to decreased correlation (Pearson r = 0.575, p = 0.032 versus r = 0.496, p = 0.070, respectively). When we compared the travel and local infection rates for Cuba with versus without the enhanced surveillance, we once again found that case exclusion led to decreased correlation (Pearson r = 0.516, p = 0.059 versus r = 0.458, p = 0.1, respectively). Therefore, we determined that including all the data would increase our estimates of the outbreak sizes in Cuba, and chose to use only cases captured by traditional reporting to not overestimate these local outbreaks.
Yearly travel infection rates from all exposure (origin) and reporting (destination) combinations were calculated by the number of travel-associated cases per 100,000 airline passenger journeys (from origin to destination/year). Exposure-reporting combinations that accounted for less than 80 imported cases were not included in this analysis; therefore, only data from Cuba, Dominican Republic, Haiti, Jamaica, and Puerto Rico are included. Air travel data were obtained as detailed below.
Air passenger volumes
We collected air passenger volumes to calculate dengue travel infection rates. We obtained the number of passengers traveling by air from Cuba, the Dominican Republic, Haiti, Jamaica, and Puerto Rico that disembarked in the US port of entry in Florida from 2009 to 2022 as international and domestic non-stop segment traffic reports (T100) from the Office of Data, Analytics, and Technology (DAT), Division of Global Migration Health (DGMH). The flight travel volume data were provided by OAG Aviation Worldwide Ltd. OAG DOT Analyser, T100 International and Domestic Segment Traffic Reports, Version 2.6.1 2023 ( https://analytics.oag.com/analyser-client/home ; accessed May 16, 2023). United States Department of Transportation (DOT) data includes all commercial chartered and military flights arriving in airports in the United States and includes origin and destination. One limitation of DOT data is that it does not include connecting flight information. However, as this would lead to a potential over-estimation of total travelers and, therefore, potentially an under-estimation of travel infection rates, we felt this was reasonable.
Cruise and boat travel data were not included. There were very few infections linked with boat travel in our dataset, even between Cuba and Florida. There were also very few infections linked to cruise travel. It may be that these cases are more likely to be tourists who are subsequently diagnosed elsewhere (and just visiting Florida for the cruise departure) or that they may have different risk behaviors compared to air travelers.
Local cases and infection rates
PAHO is the primary source for information regarding dengue spread in the Americas, with data on suspected and confirmed cases for countries and territories within the Americas with local transmission 7 . We obtained yearly dengue case counts in Cuba, Dominican Republic, Haiti, Jamaica, and Puerto Rico from 2009 to 2022, as well as local dengue infection rates (suspected and confirmed dengue cases per 100,000 population). Infection rates were used as an estimate for infection likelihood to investigate sources of dengue introductions.
Estimated local dengue cases
We constructed a negative binomial regression model to predict local infection rates from the travel infection rates due to evidence of overdispersion in the initial Poisson regression model that was tested using the performance package in R . Our final model can be seen below:
Where \({Y}_{t}\) is the number of cases at time period t , r is a measure of overdispersion in the data and \({\lambda }_{t}\) is the expected number of cases at time period t . In the regression equation, \({{pop}}_{t}\) is the country population at time t as an offset term for expected number cases to get an infection rate. We used two data types: locally acquired dengue cases by country and travel-associated dengue cases by country of origin to inform estimates of local infection rates in Cuba and Haiti on a scale comparable to local infections in the other three locations. A logarithmic relationship between travel and local infection rates was specified to account for high travel infections ( \({Travel\; Infection\; Rate}\) ), particularly in Cuba. The value of 0.02 was added to avoid computational problems when travel infection rates equaled 0 and was selected via sensitivity analysis (lowest AIC score). Because we theorized that countries and territories with a higher GDP (obtained from https://worldpopulationreview.com/countries ) would be able to devote more resources to finding and reporting local dengue cases, we created the variable \({GDPcat}\) by income, with lower-middle as GDP <$12,535 and upper as >$12,535. This ultimately placed Puerto Rico in the upper category and stratified the other four countries in the lower category. To account for the temporal trend in the outcome, we included a \({Year}\) as a linear predictor in the model. We tested for autocorrelation in Puerto Rico, Jamaica, and the Dominican Republic separately using the Durbin–Watson test and found no significant autocorrelation ( p = 0.90, 0.91, and 0.89, respectively). In addition, we tested a mixed model allowing for a country- or territory-specific random GDP effect that ultimately performed worse (AIC 752 compared to 749 for the fixed model) R code and data are available at: https://github.com/grubaughlab/2023_paper_DENV-travelers
Dengue virus sequencing
Patient serum samples were selected for study inclusion according to Ct values from prior FDOH Trioplex and serotype-specific PCR diagnostics and/or volume availability. In general, samples with a Ct value < 35 were aliquoted to provide 160 µL per sample. Lesser volume samples were included if Ct value < 25. All samples were de-identified to maintain patient privacy and blind the study.
140 µL or less of each sample was manually extracted using the QIAamp Viral RNA Mini Kit (Qiagen, Germantown, MD) following the protocol outlined in the manual unless otherwise specified. Linear acrylamide (Invitrogen by Thermo Fisher Scientific, Waltham, MA) was substituted, 1 µL of 5 mg/mL per sample, in place of the kit provided carrier RNA. Each sample was eluted in 60 µL of ultrapure, nuclease-free water (Invitrogen by Thermo Fisher Scientific, Waltham, MA) and stored at −80 °C. Sample extracts were kept frozen on dry ice during transport and shipped to collaborators for subsequent sequencing.
Dengue virus RNA was sequenced using a pan-serotype, highly multiplexed PCR approach called ‘DengueSeq’ (a derivative of PrimalSeq 64 ). A detailed protocol, including the DENV1-4 primer schemes, is available 65 , 66 . In brief, we prepared libraries using the Illumina COVIDSeq test (RUO version) with the DENV1-4 primers, and pooled libraries were sequenced on the Illumina NovaSeq (paired-end 150), targeting 1 million reads per individual library. Consensus genomes were generated at a depth of coverage of 20X and a minimum frequency threshold of 0.75 using our DengueSeq bioinformatics pipeline 67 , which includes iVar 68 . All DENV genomes and sequencing data are available on BioProject PRJNA951702.
Phylogenetic analyses
To account for differences in sampling intensity globally and to highlight dynamics within the Caribbean; we performed sample selection on the global background dataset. We used all sequences sampled from the Caribbean and then took up to 10 sequences per country or territory per year per serotype, with a strict coverage cut-off applied to all sequences of 70% of genome coverage. We then aligned these sequences with MAFFT v.7.490 69 and manually curated them using Geneious v 2022.1.1 ( https://www.geneious.com ). We generated an initial Maximum Likelihood (ML) tree for each serotype separately using the HKY substitution model 70 in IQTree v2.1.4 71 . We put this ML tree into TempEST 72 to assess the molecular clock signal and to identify molecular clock outliers, which were then removed 72 . Any remaining sylvatic sequences were also removed. The final dataset sizes therefore were DENV-1 n = 1095, DENV-2 n = 1406, DENV-3 n = 839, and DENV-4 n = 345.
In the interests of computational efficiency, we split the analysis into topology estimation and phylogeographic inference. We began by estimating the topology in BEAST v1.10.5 73 using a generalized time reversible (GTR) model 74 , a gamma rate heterogeneity model with four categories 75 , and a relaxed molecular clock 75 as in ref. 41 . The mean clock rate of each tree, using a CTMC scale prior on the mean and an exponential prior on the standard deviation, and their 95% HPDs are: 6.61 × 10 −4 (5.81 × 10 −4 to 6.41 × 10 −4 ), 8.20 × 10 −4 (7.78 × 10 −4 to 8.65 × 10 −4 ), 6.69 × 10 −4 (6.28 × 10 −4 to 7.05 × 10 −4 ) and 7.68 × 10 −4 (6.34 × 10 −4 to 8.94 ×10 −4 ) substitutions/site/year for DENV-1 to -4, respectively.
We used a Skygrid coalescent model 76 with a Hamiltonian Monte Carlo (HMC) operator 77 . We defined the gridpoints for effective population size estimation externally to be at the start of each year, every year until 2000, and then every 25 years until 1900 for DENV-1 and DENV-3, and every 50 years until 1710 for DENV-2 and every 50 years until 1750 for DENV-4. These gridpoints aimed to balance resolution in more recent years against computational efficiency, and final dates were placed slightly before the estimated roots of the trees, as described in 78 .
The length and number of chains required for convergence and sufficient ESS values depended on the serotype but ranged between two and four chains of 100–600 m states with 10–60% removed for burn-in.
From each of these analyses, we randomly sampled 500 trees from the post-burn-in posterior distribution to use as an empirical tree distribution to infer the geographic spread of each dengue virus serotype. We grouped together locations of sequences, starting with WHO regions (Africa, Europe, eastern Mediterranean, Southeast Asia, and western Pacific). The Americas were further split into South America, Central America plus Mexico, and the rest of North America. We also split the Caribbean into Jamaica, Haiti, Cuba, Dominican Republic, Puerto Rico, and other Caribbean. In total, there were 14 regions for DENV-1 and DENV-2, 12 for DENV-4, and 10 for DENV-3. Details of the number of sequences for each region can be found in Supplementary Table S1 . We performed a discrete asymmetric phylogeographic analysis using these regions and a CTMC prior 79 . Each serotype analysis was run for two chains of 10 m states, each with 10% removed for burn-in. Assessment of convergence for every analysis was performed using Tracer 78 .
Introductions were inferred using a custom Python script. We defined an introduction as the oldest node on a branch which transitioned into the territory/region of interest and counted all downstream nodes as part of the same introduction regardless of further exports/reintroductions. The time of introduction was taken conservatively, as the time of the transition node itself, and should be interpreted as the latest approximate time that the introduction could have occurred (i.e. the introduction could have occurred earlier, but not later). The XML files are available at: https://github.com/grubaughlab/2023_paper_DENV-travelers .
Reporting summary
Further information on research design is available in the Nature Portfolio Reporting Summary linked to this article.
Data availability
All DENV genomes and sequencing data generated in this study are available on BioProject PRJNA951702. Alignments provided in our data repository include all GenBank accession numbers for any publicly available sequences that were analyzed in this study ( https://github.com/grubaughlab/2023_paper_DENV-travelers ). The travel surveillance data is available in the Supplementary Information file. The air passenger data used in this study are proprietary and were purchased from OAG Aviation Worldwide Ltd. These data were used under the United States Centers for Disease Control and Prevention license for the current study, and so are not publicly available. The authors are available to share the air passenger data upon request and with the permission of OAG Aviation Worldwide Ltd.
Code availability
All code and model results are available at: https://github.com/grubaughlab/2023_paper_DENV-travelers .
Kimberlin, D. W., Barnett, E., Lynfield, R. & Sawyer, M. H. Red Book 2021: Report of the Committee on Infectious Diseases . (American Academy of Pediatrics, 2021).
Guzman, M. G. & Harris, E. Dengue. Lancet 385 , 453–465 (2015).
Article PubMed Google Scholar
Bhatt, S. et al. The global distribution and burden of dengue. Nature 496 , 504–507 (2013).
Article ADS CAS PubMed PubMed Central Google Scholar
Waggoner, J. J. et al. Homotypic Dengue Virus Reinfections in Nicaraguan Children. J. Infect. Dis. 214 , 986–993 (2016).
Article PubMed PubMed Central Google Scholar
Christie, C. D. C., Lue, A. M. & Melbourne-Chambers, R. H. Dengue, chikungunya and zika arbovirus infections in Caribbean children. Curr. Opin. Pediatr. 35 , 155–165 (2023).
Verhagen, L. M. & de Groot, R. Dengue in children. J. Infect. 69 , S77–S86 (2014).
PAHO. Dengue https://www3.paho.org/data/index.php/en/mnu-topics/indicadores-dengue-en.html (2022).
Brathwaite Dick, O. et al. The history of dengue outbreaks in the Americas. Am. J. Trop. Med. Hyg. 87 , 584–593 (2012).
Tapia-Conyer, R., Betancourt-Cravioto, M. & Méndez-Galván, J. Dengue: an escalating public health problem in Latin America. Paediatr. Int. Child Health 32 , 14–17 (2012).
Weaver, S. C. Arrival of chikungunya virus in the new world: prospects for spread and impact on public health. PLoS Negl. Trop. Dis. 8 , e2921 (2014).
Fischer, M. & Staples, J. E., Arboviral Diseases Branch, National Center for Emerging and Zoonotic Infectious Diseases, CDC. Notes from the field: chikungunya virus spreads in the Americas - Caribbean and South America, 2013-2014. MMWR Morb. Mortal. Wkly. Rep. 63 , 500–501 (2014).
PubMed PubMed Central Google Scholar
Santiago, G. A. et al. Tracing the Origin, Spread, and Molecular Evolution of Zika Virus in Puerto Rico, 2016-2017. Emerg. Infect. Dis. 27 , 2971–2973 (2021).
Article CAS PubMed PubMed Central Google Scholar
Faria, N. R. et al. Establishment and cryptic transmission of Zika virus in Brazil and the Americas. Nature 546 , 406–410 (2017).
Worsley-Tonks, K. E. L. et al. Strengthening global health security by improving disease surveillance in remote rural areas of low-income and middle-income countries. Lancet Glob. Health 10 , e579–e584 (2022).
Magalhães, A. R. et al. Neglected tropical diseases risk correlates with poverty and early ecosystem destruction. Infect. Dis. Poverty 12 , 32 (2023).
Da Conceição Araújo, D. et al. Determining the association between dengue and social inequality factors in north-eastern Brazil: A spatial modelling. Geospat. Health 15 , 71–80 (2020).
do Carmo, R. F., Silva Júnior, J. V. J., Pastor, A. F. & de Souza, C. D. F. Spatiotemporal dynamics, risk areas and social determinants of dengue in Northeastern Brazil, 2014-2017: an ecological study. Infect. Dis. Poverty 9 , 153 (2020).
Grubaugh, N. D. et al. Travel Surveillance and Genomics Uncover a Hidden Zika Outbreak during the Waning Epidemic. Cell 178 , 1057–1071.e11 (2019).
Leder, K. et al. Zika beyond the Americas: Travelers as sentinels of Zika virus transmission. A GeoSentinel analysis, 2012 to 2016. PLoS One 12 , e0185689 (2017).
Wilder-Smith, A., Chang, C. R. & Leong, W. Y. Zika in travellers 1947-2017: a systematic review. J. Travel Med . 25 , tay044 (2018).
Hamer, D. H. et al. Travel-Associated Zika Virus Disease Acquired in the Americas Through February 2016: A GeoSentinel Analysis. Ann. Intern. Med. 166 , 99–108 (2017).
Harvey, K. et al. Surveillance for travel-related disease–GeoSentinel Surveillance System, United States, 1997-2011. MMWR Surveill. Summ. 62 , 1–23 (2013).
PubMed Google Scholar
Leder, K. et al. GeoSentinel surveillance of illness in returned travelers, 2007-2011. Ann. Intern. Med. 158 , 456–468 (2013).
Angelo, K. M. et al. Zika among international travellers presenting to GeoSentinel sites, 2012-2019: implications for clinical practice. J. Travel Med. 27 , taaa222 (2020).
Article Google Scholar
Osman, S. & Preet, R. Dengue, chikungunya and Zika in GeoSentinel surveillance of international travellers: a literature review from 1995 to 2020. J. Travel Med . 27 , taaa222 (2020).
Fraser, C. et al. Pandemic potential of a strain of influenza A (H1N1): early findings. Science 324 , 1557–1561 (2009).
The World Bank in the Caribbean. World Bank https://www.worldbank.org/en/country/caribbean/overview (2023).
Wilson, M. E. et al. Fever in returned travelers: results from the GeoSentinel Surveillance Network. Clin. Infect. Dis. 44 , 1560–1568 (2007).
Fink, D., Wani, R. S. & Johnston, V. Fever in the returning traveller. BMJ 360 , j5773 (2018).
Marks, M., Johnston, V. & Brown, M. 150 - Fever in the Returned Traveler. In Hunter’s Tropical Medicine and Emerging Infectious Diseases, 10th ed. (eds Ryan, E. T., Hill, D. R., Solomon, T., Aronson, N. E. & Endy, T. P.) 1077–1086 (Elsevier, London, 2020).
Rey, J. R. Dengue in Florida (USA). Insects 5 , 991–1000 (2014).
Díaz-Menéndez, M. et al. Dengue outbreak amongst travellers returning from Cuba-GeoSentinel surveillance network, January-September 2022. J. Travel Med . 30 , taac139 (2023).
Naveca, F. G. et al. Reemergence of dengue virus serotype 3, Brazil, 2023. bioRxiv https://doi.org/10.1101/2023.05.03.23288351 (2023).
Torres, J. R., Orduna, T. A., Piña-Pozas, M., Vázquez-Vega, D. & Sarti, E. Epidemiological Characteristics of Dengue Disease in Latin America and in the Caribbean: A Systematic Review of the Literature. J. Trop. Med. 2017 , 8045435 (2017).
Gómez-Dantés, H. & Willoquet, J. R. Dengue in the Americas: challenges for prevention and control. Cad. Saude Publica 25 , S19–S31 (2009).
Gossner, C. M. et al. Dengue virus infections among European travellers, 2015 to 2019. Euro Surveill . 27 , 2001937 (2022).
Ryff, K. R. et al. Epidemiologic Trends of Dengue in U.S. Territories, 2010-2020. MMWR Surveill. Summ. 72 , 1–12 (2023).
Katzelnick, L. C. et al. Antigenic evolution of dengue viruses over 20 years. Science 374 , 999–1004 (2021).
Zhang, C. et al. Clade replacements in dengue virus serotypes 1 and 3 are associated with changing serotype prevalence. J. Virol. 79 , 15123–15130 (2005).
Adams, B. et al. Cross-protective immunity can account for the alternating epidemic pattern of dengue virus serotypes circulating in Bangkok. Proc. Natl Acad. Sci. USA 103 , 14234–14239 (2006).
Brito, A. F. et al. Lying in wait: the resurgence of dengue virus after the Zika epidemic in Brazil. Nat. Commun. 12 , 2619 (2021).
Jones, F. K. et al. Introduction and Spread of Dengue Virus 3, Florida, USA, May 2022-April 2023. Emerg. Infect. Dis . 30 , 376–379 (2024).
Santos, L. L. M. et al. Dengue, chikungunya, and Zika virus infections in Latin America and the Caribbean: a systematic review. Rev. Panam. Salud Publica 47 , e34 (2023).
FDOH. Dengue Fever https://www.floridahealth.gov/diseases-and-conditions/dengue/ (2022).
Guzman, M. G. & Kouri, G. Dengue and dengue hemorrhagic fever in the Americas: lessons and challenges. J. Clin. Virol. 27 , 1–13 (2003).
MMWR. Dengue type 3 infection—Nicaragua and Panamá, October–November, 1994 . 21–24 (MMWR, 1995).
Guzmán, M. G., Huelva, G., Saenz, E. & Quiroz, E. Reintroduccion del dengue 3 en las Americas: 1994-1996. Arch. Venez. Med. Trop. Parasitol. Med . 2 , 8–19 (1998)
Figueroa, R. & Ramos, C. Dengue virus (serotype 3) circulation in endemic countries and its reappearance in America. Arch. Med. Res. 31 , 429–430 (2000).
Article CAS PubMed Google Scholar
Briseño-García, B. et al. Potential risk for dengue hemorrhagic fever: the isolation of serotype dengue-3 in Mexico. Emerg. Infect. Dis. 2 , 133–135 (1996).
Aogo, R. A. et al. Effects of boosting and waning in highly exposed populations on dengue epidemic dynamics. Sci. Transl. Med . 15 , eadi1734 (2023).
López, L., Paul, R. E., Cao-Lormeau, V.-M. & Rodó, X. Considering waning immunity to better explain dengue dynamics. Epidemics 41 , 100630 (2022).
Forshey, B. M., Stoddard, S. T. & Morrison, A. C. Dengue Viruses and Lifelong Immunity: Reevaluating the Conventional Wisdom. J. Infect. Dis. 214 , 979–981 (2016).
Salje, H. et al. Reconstruction of antibody dynamics and infection histories to evaluate dengue risk. Nature 557 , 719–723 (2018).
OhAinle, M. et al. Dynamics of dengue disease severity determined by the interplay between viral genetics and serotype-specific immunity. Sci. Transl. Med. 3 , 114ra128 (2011).
Santiago, G. A. et al. Reemergence and decline of dengue virus serotype 3 in Puerto Rico. J. Infect. Dis. 206 , 893–901 (2012).
L’Azou, M. et al. Dengue seroprevalence in the French West Indies: a prospective study in adult blood donors. Am. J. Trop. Med. Hyg. 92 , 1137–1140 (2015).
Loroño-Pino, M. A. et al. Common occurrence of concurrent infections by multiple dengue virus serotypes. Am. J. Trop. Med. Hyg. 61 , 725–730 (1999).
Sirisena, N. et al. Concurrent dengue infections: Epidemiology & clinical implications. Indian J. Med. Res. 154 , 669–679 (2021).
Article PubMed Central Google Scholar
Andrade, E. H. P. et al. Spatial-Temporal Co-Circulation of Dengue Virus 1, 2, 3, and 4 Associated with Coinfection Cases in a Hyperendemic Area of Brazil: A 4-Week Survey. Am. J. Trop. Med. Hyg. 94 , 1080–1084 (2016).
Sharp, T. M., Ryff, K. R., Santiago, G. A., Margolis, H. S. & Waterman, S. H. Lessons Learned from Dengue Surveillance and Research, Puerto Rico, 1899-2013. Emerg. Infect. Dis. 25 , 1522–1530 (2019).
Boyette, C. US Coast Guard returns 273 migrants intercepted off Florida Coast to Cuba . (CNN, 2023).
FL DOH. Mosquito-Borne Disease Surveillance http://www.floridahealth.gov/diseases-and-conditions/mosquito-borne-diseases/surveillance.html (2022).
Santiago, G. A. et al. Analytical and clinical performance of the CDC real time RT-PCR assay for detection and typing of dengue virus. PLoS Negl. Trop. Dis. 7 , e2311 (2013).
Quick, J. et al. Multiplex PCR method for MinION and Illumina sequencing of Zika and other virus genomes directly from clinical samples. Nat. Protoc. 12 , 1261–1276 (2017).
Vogels, C. et al. DengueSeq: A pan-serotype whole genome amplicon sequencing protocol for dengue virus. protocols.io . https://www.protocols.io/view/dengueseq-a-pan-serotype-whole-genome-amplicon-seq-kqdg39xxeg25/v2 . https://doi.org/10.17504/protocols.io.kqdg39xxeg25/v1 (2022).
Vogels, C. B. F. et al. DengueSeq: A pan-serotype whole genome amplicon sequencing protocol for dengue virus. medRxiv https://doi.org/10.1101/2023.10.13.23296997 (2023).
Hill, V., Chaguza, C. & Lab, G. DENV_pipeline. https://github.com/grubaughlab/DENV_pipeline (2023).
Grubaugh, N. D. et al. An amplicon-based sequencing framework for accurately measuring intrahost virus diversity using PrimalSeq and iVar. Genome Biol. 20 , 8 (2019).
Katoh, K. & Standley, D. M. MAFFT multiple sequence alignment software version 7: improvements in performance and usability. Mol. Biol. Evol. 30 , 772–780 (2013).
Hasegawa, M., Kishino, H. & Yano, T. Dating of the human-ape splitting by a molecular clock of mitochondrial DNA. J. Mol. Evol. 22 , 160–174 (1985).
Article ADS CAS PubMed Google Scholar
Minh, B. Q. et al. IQ-TREE 2: New Models and Efficient Methods for Phylogenetic Inference in the Genomic Era. Mol. Biol. Evol. 37 , 1530–1534 (2020).
Rambaut, A., Lam, T. T., Max Carvalho, L. & Pybus, O. G. Exploring the temporal structure of heterochronous sequences using TempEst (formerly Path-O-Gen). Virus Evol. 2 , vew007 (2016).
Suchard, M. A. et al. Bayesian phylogenetic and phylodynamic data integration using BEAST 1.10. Virus Evol. 4 , vey016 (2018).
Tarvare, S. Some probabilistic and statistical problems in the analysis of DNA sequences. In Some mathematical question in biology-DNA sequence analysis (American Mathematical Society, 1986).
Yang, Z. Maximum likelihood phylogenetic estimation from DNA sequences with variable rates over sites: approximate methods. J. Mol. Evol. 39 , 306–314 (1994).
Gill, M. S. et al. Improving Bayesian population dynamics inference: a coalescent-based model for multiple loci. Mol. Biol. Evol. 30 , 713–724 (2013).
Baele, G., Gill, M. S., Lemey, P. & Suchard, M. A. Hamiltonian Monte Carlo sampling to estimate past population dynamics using the skygrid coalescent model in a Bayesian phylogenetics framework. Wellcome Open Res. 5 , 53 (2020).
Hill, V. & Baele, G. Bayesian Estimation of Past Population Dynamics in BEAST 1.10 Using the Skygrid Coalescent Model. Mol. Biol. Evol. 36 , 2620–2628 (2019).
Ferreira, M. A. R. & Suchard, M. A. Bayesian analysis of elapsed times in continuous-time Markov chains. Can. J. Stat. 36 , 355–368 (2008).
Article MathSciNet Google Scholar
Download references
Acknowledgements
We acknowledge S. Taylor and P. Jack for technical discussions. This publication was made possible by the National Institute of Allergy and Infectious Diseases of the National Institutes of Health (NIH) under Award Number DP2AI176740 (NDG), CTSA Grant Number UL1 TR001863 from the National Center for Advancing Translational Science, a component of the NIH (CBFV), the NIH T32AI055403 (AS), National Science Foundation (NSF) Graduate Research Fellowship under Grant No. DGE-2139841 (AS), The Dominican Ministry of Higher Education and Technology Fund (FONDOCYT-2022-1B3-069), the National Institute of General Medical Sciences R21GM142011 (SFM), and through a Cooperative Agreement between the US Centers for Disease Control and Prevention (CDC) and the International Society of Travel Medicine (ISTM) (Federal Award Number: 1 U01CK000632-01-00). The findings and conclusions in this report are those of the author(s) and do not necessarily represent the official position of the CDC, NIH, or FDOH.
Author information
These authors contributed equally: Emma Taylor-Salmon, Verity Hill, Lauren M. Paul, Robert T. Koch.
These authors jointly supervised this work: Chantal B. F. Vogels, Andrea Morrison, Scott F. Michael, Nathan D. Grubaugh.
Authors and Affiliations
Department of Pediatrics, Yale School of Medicine, New Haven, CT, USA
Emma Taylor-Salmon
Department of Epidemiology of Microbial Diseases, Yale School of Public Health, New Haven, CT, USA
Emma Taylor-Salmon, Verity Hill, Robert T. Koch, Mallery I. Breban, Chrispin Chaguza, Afeez Sodeinde, Chantal B. F. Vogels & Nathan D. Grubaugh
Department of Biological Sciences, College of Arts and Sciences, Florida Gulf Coast University, Fort Myers, FL, USA
Lauren M. Paul & Scott F. Michael
Department of Biostatistics, Yale School of Public Health, New Haven, CT, USA
Joshua L. Warren
Public Health Modeling Unit, Yale School of Public Health, New Haven, CT, USA
Joshua L. Warren & Nathan D. Grubaugh
Bureau of Public Health Laboratories, Division of Disease Control and Health Protection, Florida Department of Health, Tampa, FL, USA
Sylvia Bunch, Natalia Cano, Marshall Cone, Sarah Eysoldt, Alezaundra Garcia, Nicadia Gilles, Andrew Hagy, Lea Heberlein, Rayah Jaber & Elizabeth Kassens
Bureau of Public Health Laboratories, Division of Disease Control and Health Protection, Florida Department of Health, Jacksonville, FL, USA
Pamela Colarusso & Amanda Davis
Florida Department of Health in Miami-Dade County, Miami, FL, USA
Samantha Baudin, Edhelene Rico & Álvaro Mejía-Echeverri
Bureau of Epidemiology, Division of Disease Control and Health Protection, Florida Department of Health, Tallahassee, FL, USA
Blake Scott, Danielle Stanek, Rebecca Zimler & Andrea Morrison
Division of Vector-Borne Diseases, Centers for Disease Control and Prevention, San Juan, Puerto Rico
Jorge L. Muñoz-Jordán, Gilberto A. Santiago, Laura E. Adams & Gabriela Paz-Bailey
Office of Data, Analytics, and Technology, Division of Global Migration Health, Centers for Disease Control and Prevention, Atlanta, GA, USA
Melanie Spillane & Volha Katebi
Bureau for Global Health, United States Agency for International Development, Arlington, VA, USA
Melanie Spillane
Instituto de Medicina Tropical & Salud Global, Universidad Iberoamericana, UNIBE Research Hub, Santo Domingo, Dominican Republic
Robert Paulino-Ramírez, Sayira Mueses, Armando Peguero & Nelissa Sánchez
National Referral Unit for Tropical Diseases, Infectious Diseases Department, CIBER de Enfermedades Infecciosas, IRYCIS, Hospital Ramón y Cajal, Universidad de Alcalá, Madrid, Spain
Francesca F. Norman
Microbiology Department, Hospital Ramón y Cajal, Instituto Ramón y Cajal de Investigación Sanitaria (IRYCIS), CIBER de Epidemiologia y Salud Publica (CIBERESP), Madrid, Spain
Juan-Carlos Galán
Department of Infectious Tropical Diseases and Microbiology, IRCCS Sacro Cuore Don Calabria Hospital, Negrar, Verona, Italy
Ralph Huits
Department of Global Health, Boston University School of Public Health, Section of Infectious Diseases, Boston University School of Medicine, Center for Emerging Infectious Disease Policy and Research, Boston University, and National Emerging Infectious Disease Laboratory, Boston, MA, USA
Davidson H. Hamer
Yale Institute for Global Health, Yale University, New Haven, CT, USA
Chantal B. F. Vogels & Nathan D. Grubaugh
Department of Ecology and Evolutionary Biology, Yale University, New Haven, CT, USA
Nathan D. Grubaugh
You can also search for this author in PubMed Google Scholar
Contributions
E.T.S, C.B.F.V, A.M., S.F.M., and N.D.G. designed the study. E.T.S., V.H., L.M.P., and R.T.K. wrote the paper. L.M.P., A.M., S.Bu., N.C., M.C., S.E., A.G., N.G., A.H., L.H., R.J., E.K., P.C., A.D., S.Ba., E.R., A.M.E., B.S., D.S., R.Z., R.P.R, S.M., A.P., N.S., F.F.N., J.C.G., J.L.M.J., G.A.S, L.E.A, G.P.B., R.H., and D.H.H. collected clinical samples and data. M.I.B, C.C., A.S., V.H., and C.B.F.V. performed the sequencing and bioinformatic analysis. M.S. and V.K. provided travel data. E.T.S., R.T.K., V.H., and J.L.W. performed statistical and phylogenetic analyses. N.D.G, C.B.F.V, A.M. and S.F.M supervised the project. All authors reviewed and approved the final version of the manuscript.
Corresponding authors
Correspondence to Emma Taylor-Salmon , Andrea Morrison , Scott F. Michael or Nathan D. Grubaugh .
Ethics declarations
Competing interests.
N.D.G. is a paid consultant for BioNTech for work unrelated to this manuscript. All other authors declare no competing interests.
Peer review
Peer review information.
Nature Communications thanks the anonymous reviewer(s) for their contribution to the peer review of this work. A peer review file is available.
Additional information
Publisher’s note Springer Nature remains neutral with regard to jurisdictional claims in published maps and institutional affiliations.
Supplementary information
Supplementary information, peer review file, reporting summary, rights and permissions.
Open Access This article is licensed under a Creative Commons Attribution 4.0 International License, which permits use, sharing, adaptation, distribution and reproduction in any medium or format, as long as you give appropriate credit to the original author(s) and the source, provide a link to the Creative Commons licence, and indicate if changes were made. The images or other third party material in this article are included in the article’s Creative Commons licence, unless indicated otherwise in a credit line to the material. If material is not included in the article’s Creative Commons licence and your intended use is not permitted by statutory regulation or exceeds the permitted use, you will need to obtain permission directly from the copyright holder. To view a copy of this licence, visit http://creativecommons.org/licenses/by/4.0/ .
Reprints and permissions
About this article
Cite this article.
Taylor-Salmon, E., Hill, V., Paul, L.M. et al. Travel surveillance uncovers dengue virus dynamics and introductions in the Caribbean. Nat Commun 15 , 3508 (2024). https://doi.org/10.1038/s41467-024-47774-8
Download citation
Received : 11 November 2023
Accepted : 11 April 2024
Published : 25 April 2024
DOI : https://doi.org/10.1038/s41467-024-47774-8
Share this article
Anyone you share the following link with will be able to read this content:
Sorry, a shareable link is not currently available for this article.
Provided by the Springer Nature SharedIt content-sharing initiative
By submitting a comment you agree to abide by our Terms and Community Guidelines . If you find something abusive or that does not comply with our terms or guidelines please flag it as inappropriate.
Quick links
- Explore articles by subject
- Guide to authors
- Editorial policies
Sign up for the Nature Briefing newsletter — what matters in science, free to your inbox daily.

What is Dengue?
Dengue viruses are spread to people through the bite of an infected Aedes species ( Ae. aegypti or Ae. albopictus ) mosquito. Almost half of the world’s population, about 4 billion people, live in areas with a risk of dengue . Dengue is often a leading cause of illness in areas with risk.
Areas with Risk of Dengue
Dengue outbreaks are occurring in many countries of the world. Protect yourself from mosquito bites .
Dengue Vaccine
A dengue vaccine is approved for use in children aged 9 to 16 years with laboratory-confirmed previous dengue virus infection and living in areas where dengue is endemic (common). Endemic areas include some U.S. territories and freely associated states. The vaccine is not approved for use in U.S. travelers who are visiting but not living in an area where dengue is common.
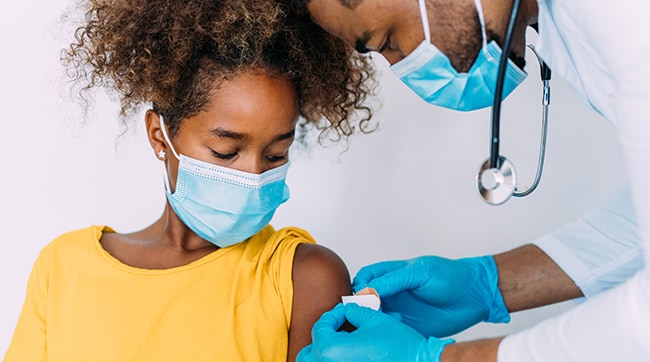
A dengue vaccine is recommended in areas where dengue is endemic (common).

Mosquitoes spread dengue to people through bites
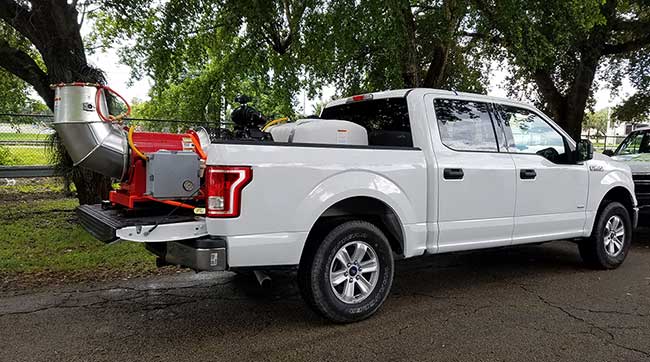
Truck spraying can help control mosquitoes
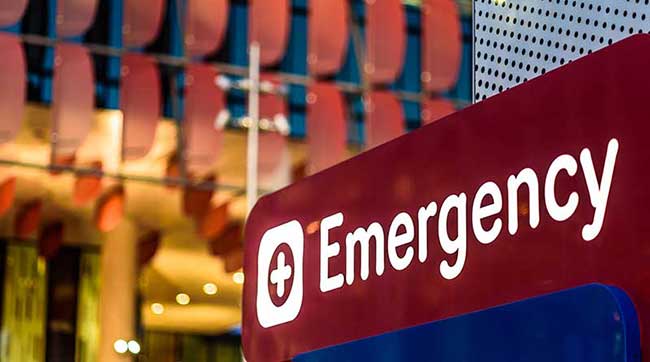
Severe dengue is a medical emergency
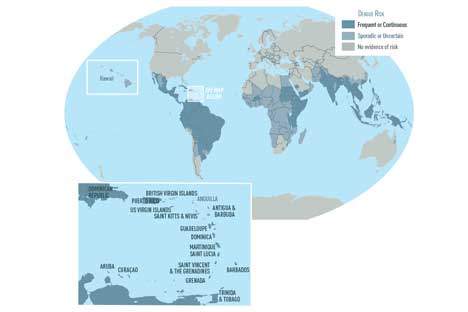
Exit Notification / Disclaimer Policy
- The Centers for Disease Control and Prevention (CDC) cannot attest to the accuracy of a non-federal website.
- Linking to a non-federal website does not constitute an endorsement by CDC or any of its employees of the sponsors or the information and products presented on the website.
- You will be subject to the destination website's privacy policy when you follow the link.
- CDC is not responsible for Section 508 compliance (accessibility) on other federal or private website.
- Open access
- Published: 22 April 2024
Dengue virus pathogenesis and host molecular machineries
- Saumya Sinha 1 ,
- Kinjal Singh 1 ,
- Y. S. Ravi Kumar 2 ,
- Riya Roy 1 ,
- Sushant Phadnis 1 ,
- Varsha Meena 1 ,
- Sankar Bhattacharyya 3 &
- Bhupendra Verma ORCID: orcid.org/0000-0003-1731-5335 1
Journal of Biomedical Science volume 31 , Article number: 43 ( 2024 ) Cite this article
572 Accesses
2 Altmetric
Metrics details
Dengue viruses (DENV) are positive-stranded RNA viruses belonging to the Flaviviridae family. DENV is the causative agent of dengue, the most rapidly spreading viral disease transmitted by mosquitoes. Each year, millions of people contract the virus through bites from infected female mosquitoes of the Aedes species. In the majority of individuals, the infection is asymptomatic, and the immune system successfully manages to control virus replication within a few days. Symptomatic individuals may present with a mild fever (Dengue fever or DF) that may or may not progress to a more critical disease termed Dengue hemorrhagic fever (DHF) or the fatal Dengue shock syndrome (DSS). In the absence of a universally accepted prophylactic vaccine or therapeutic drug, treatment is mostly restricted to supportive measures. Similar to many other viruses that induce acute illness, DENV has developed several ways to modulate host metabolism to create an environment conducive to genome replication and the dissemination of viral progeny. To search for new therapeutic options, understanding the underlying host-virus regulatory system involved in various biological processes of the viral life cycle is essential. This review aims to summarize the complex interaction between DENV and the host cellular machinery, comprising regulatory mechanisms at various molecular levels such as epigenetic modulation of the host genome, transcription of host genes, translation of viral and host mRNAs, post-transcriptional regulation of the host transcriptome, post-translational regulation of viral proteins, and pathways involved in protein degradation.
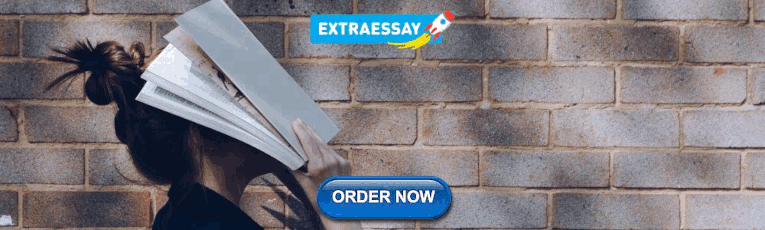
Introduction
Dengue virus (DENV), a positive-sense (+) single-stranded RNA virus belonging to the Flavivirus family, has spherical-shaped envelope virion particles with surface proteins arranged in icosahedral symmetry [ 1 , 2 ]. The viral genome is about 11 kb long and is translated directly upon entering host cells by the protein synthesis machinery, producing both viral structural and non-structural proteins. The DENV genome synthesizes three structural proteins (Capsid, Membrane, Envelope) which form part of a mature virion particle, and seven non-structural proteins (NS1, NS2A, NS2B, NS3, NS4A, NS4B, and NS5), which helps in replication [ 3 ]. The RNA genome and the capsid protein interact to form a complex, while other structural proteins form part of the virion envelope [ 4 ]. Although the NS proteins are absent within the virion, they assist in virus replication and evasion of the immune system within an infected cell [ 3 ]. The structural organization and life cycle of DENV is shown in Fig. 1 .
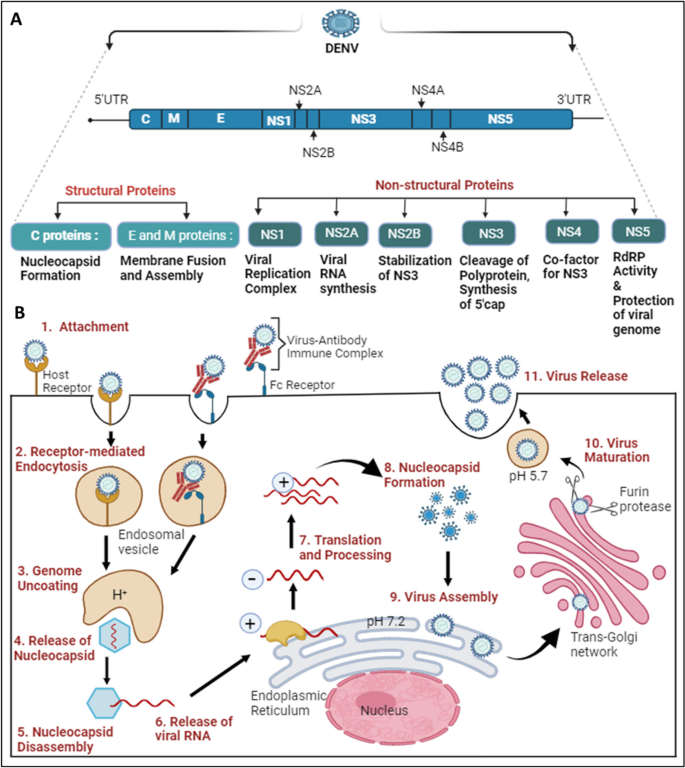
Structural organization and life cycle of DENV: A The Dengue virus genome comprises 5’UTR, ORF, and 3’UTR. The ORF translates into a polyprotein, which is further processed into three structural proteins: C (Capsid), E (Envelope), and M (Membrane), and seven non-structural proteins (NS1, NS2A, NS2B, NS3, NS4A, NS4B, NS5). B The initiation of the DENV replication cycle occurs with the entry into the cell via various host cell receptors or through the Fc region of the virus-antibody immune complex, which attaches to Fc receptors present on the target host cell. 1. DENV attaches to host cell receptors and enters the cell. 2. Internalization occurs through receptor-mediated endocytosis, forming an early endosome. 3, 4. Genome uncoating occurs as the pH decreases inside the early endosome; conformational changes take place, releasing the nucleocapsid into the cytoplasm. 5, 6. Disassembly of the nucleocapsid allows viral RNA assembly in the cytoplasm. 7. Viral RNA translocates into the Endoplasmic Reticulum, where translation results in a single polyprotein that is cleaved down by both host and viral proteases. Additionally, a translation switch results in the transcription of viral RNA employing antisense viral RNA. 8. The capsid protein encases the freshly created viral RNA to form the nucleocapsid. 9. Virus assembly occurs on the surface of the Endoplasmic Reticulum. 10. Immature viral particles are transported to the trans-Golgi network, where acidification results in conformational changes, followed by exposure to the furin protease to form mature viral particles. 11. Mature viral particles are exocytosed into the extracellular matrix, completing their replication cycle
The NS1 protein has multiple roles inside and outside the host cell; it also serves as an early indicator to diagnose and assess the level of DENV infection [ 5 , 6 ]. The protein is secreted as a hexamer into the blood circulation of patients, forming an open barrel structure with lipid molecules at the center [ 7 ]. NS1 plays a vital role in severe dengue physiopathology, specifically plasma leakage. A recent report suggested that NS1 activates macrophages via Toll-like receptor 4 (TLR4) and disrupts endothelial cells, resulting in vascular leakage as mentioned in Fig. 2 A [ 8 ]. NS3 acts as a protease using NS2B as a co-factor, cleaving the polyprotein at specific sites and host proteins that would impair dengue infection as depicted in Fig. 2 B [ 9 ]. The importance of the NS3 protein for the survival of the virus makes it a target for antiviral drugs as mentioned in Table 2 . NS4B interacts with NS3 to modulate viral infection, while NS4A indirectly assists in the viral replication process by inducing autophagy, preventing cell death, which is beneficial for viral replication [ 10 ]. NS5 is the most conserved non-structural protein having an RNA-dependent RNA polymerase (RdRp) domain. NS5 is also involved in mRNA capping due to its methyltransferase and guanylyltransferase activities [ 11 ]. The NS5 protein is predominantly located in the nucleus of infected cells, believed to suppress the host anti-viral response [ 12 ]. The DENV envelope protein is involved in attachment and host cell receptor binding for virus entry into the host cell as mentioned in Fig. 2 D [ 13 ]. The newly synthesized viral genome associates with the capsid to form a nucleocapsid, and it buds into the ER lumen together with viral E and prM proteins as shown in Fig. 2 C [ 4 ].
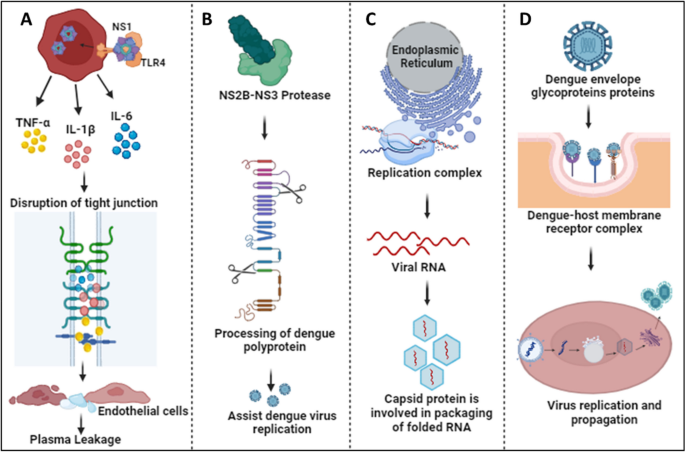
Physiological roles of DENV proteins: A The NS1 protein interacts with TLR4 and activates macrophages, resulting in cytokine release. Cytokines disrupt tight junctions and endothelial barriers, leading to plasma leakage in severe cases of dengue [ 8 ]. B NS3, together with the co-factor NS2B, participates in the processing of the dengue polyprotein, assisting in efficient virus replication [ 9 ]. C The Capsid protein helps in packaging folded RNA released from the DENV replication complex [ 4 ]. D Envelope glycoproteins attach to host receptors and form the dengue-host membrane complex, resulting in virus internalization to process its replication and propagation inside the host cell [ 13 ]
Humans and mosquitoes are the primary hosts and vectors of DENV, respectively. The dengue virus is often undetectable yet prevalent, spreading in cycles of endemic and epidemic disease [ 14 ]. In human, the infection is spread by Aedes species of mosquitoes, specifically Aedes aegypti, Aedes albopictus, and Aedes polynesiensis [ 15 ]. In addition to infected female mosquitoes transmitting the virus to their offspring through eggs, mature female mosquitoes may become infected after feeding on the blood of DENV-infected patients. After ingestion by a mosquito, the virus infects the midgut followed by propagation to other tissues, including the salivary glands. When a mosquito feeds on blood, infectious virus is injected into the skin via salivary gland secretion. Soon after the virus enters the human host’s skin, it initiate its propagation and infects the resident cells [ 16 ]. DENV circulates globally as four serotypes: DENV-1, DENV-2, DENV-3, and DENV-4 [ 17 ]. Approximately 2 to 7 days after a mosquito bite, infection with any serotype of the virus can result in Dengue fever (DF), which may quickly progress to Dengue hemorrhagic fever (DHF), and if neglected or undetected, it can lead to Dengue shock syndrome (DSS) [ 18 , 19 , 20 ]. Dengue has evolved into a serious, potentially fatal consequence for people, as there is currently no vaccine that can effectively reduce the severity of the disease caused by all serotypes, and its prevalence has been steadily increasing [ 21 ].
Whenever the dengue virus infects the human host, both the innate (interferons, complement system, etc.) and adaptive (immunoglobulins and cytotoxic T-cells) immune systems are stimulated to neutralize the virus. In severe Dengue infection, the uncontrolled generation of inflammatory cytokines, leading to phenomenon termed ‘cytokine storm’, has been principally implicated as the causal agent of fatality. Antibodies produced against DENV play a crucial role in disease outcome, as during a secondary heterotypic infection (i.e., an earlier infection by one serotype followed by the current infection by another serotype of DENV), the virus causing the secondary infection cannot be efficiently neutralized by the antibodies against the first serotype. Furthermore, a phenomenon referred to as antibody-dependent enhancement, or ADE, may be enhanced by these non-neutralizing antibodies or low amounts of neutralizing antibodies. Several vaccines have been explored to treat dengue infection, including live attenuated tetravalent vaccines and Dengvaxia. However, developing a vaccine that is effective against all four dengue serotypes remains a challenge [ 22 ]. Importantly, vaccine efficacy varies from person to person, depending on age, serotype, and serostatus [ 23 , 24 ]. Nevertheless, seronegative individuals were advised to avoid vaccination because it was reported that over time, the number of vaccine-induced antibodies decreased [ 25 ]. A potential ADE effect has been a significant restriction for the development of a prophylactic vaccine targeting DENV infection.
In spite of efforts by multiple research groups, there is still no therapeutic drug available in the market that can restrict virus replication or suppress the infection-induced ‘cytokine storm’ to reduce morbidity and/or mortality. Although various drugs, such as Chloroquine, Prednisolone, Balapiravir, Celgosivir, and Lovastatin have been tried, none of them showed promising results during clinical trials. Therefore, supportive medication is mainly used upon the diagnosis of Dengue infection. For example, treatment with paracetamol or pain medications can alleviate or reduce Dengue symptoms, such as muscle soreness, weariness, and fever. Ibuprofen and aspirin, two examples of non-steroidal anti-inflammatory medicines (NSAIDs), are commonly used, but they are not advised because they can exacerbate the prognosis of illnesses, resulting in adverse health conditions. By managing a patient’s fluid content, which is crucial for the management of severe DF, and providing proper medical care, symptoms and mortality can be reduced from more than 20% to less than 1% [ 1 , 26 ]. Notwithstanding supportive therapy, DENV infection may result in endothelial dysfunction, antibody-dependent cellular cytotoxicity (ADCC), cytokine storm (hypercytokinemia), and irregular stimulation of the complement system (CS), leading to a more severe case of Dengue [ 27 ].
Addressing the various host-viral modifications at the molecular level may provide key insights into viral evolution, viral escape mechanisms, host-virus interactions, disease consequences, and public health readiness. This information may advance our understanding of viral infections and facilitate the development of efficient preventative, diagnostic, and therapeutic approaches. The current review will specifically focus on recent advances related to the modulation of epigenomic regulation, transcription, post-transcriptional regulation, as well as the translational and post-translational machinery of the host cell.
DENV and the host epigenetic machinery
Epigenetic plays a vital role as it has the potential to regulate gene expression without altering the genetic sequences. Modulation of epigenetic regulation in the host-DENV interaction is noy yet completely understood. Recent years have seen growing evidence highlighting the pivotal role of epigenetic regulators in gene expression regulation. These regulators encompass various mechanisms such as DNA methylation, chromatin remodeling, histone modification, and regulatory RNA. A comprehensive understanding of the complex interactions among these epigenetic processes is essential for elucidating the consequences they exert on both host cells and DENV during infection. Such insights can aid in the identification of novel therapeutic targets.
Dinakaran et al. provided an overview that at the severe stage of infection, patients show symptoms characterized by inflammation and a cytokine storm resulting in oxidative stress, activating histone deacetylase (HDAC). HDAC factors such as DNA methyltransferase (DNMT) favor the silencing of promoters and slow down host gene transcription processes, as noted in Fig. 3 A. This epigenetic modification is important for investigating mental health issues related to dengue infection. Various HDAC inhibitors like valproate, quetiapine, or carbamazepine are prescribed for treatment as HDAC/Dnmt inhibitors [ 28 ]. Comprehensive transcriptome analysis identified that DENV proteins interact with host genes (CCR10, CNG7, PLXNA3) and lncRNAs (CTBP1-AS, MAFG-AS1) engaged in various biological processes, such as the immune response, inflammation, and the cell cycle, as illustrated in Fig. 3 B. The role of m6A methylation has been implicated in potentially regulating these genes/lncRNAs to enhance DENV replication, as noted in Table 1 [ 29 , 30 ]. Other flaviviruses (YFV, WNV, and ZIKV) also contain m6A methylation, indicating a conserved mechanism within this virus family [ 31 ]. An epitranscriptome analysis showed that various kinds of methylation might be used to regulate flaviviral RNA [ 32 ]. It’s interesting to note that each flavivirus has a unique methylated nucleotide composition. For example, ZIKV vRNA does not include methylated uridines m 3 Um and m 5 Um, while DENV vRNA does [ 32 ]. On the other hand, dimethylcytosines m 5 Cm and m 4 4 C are exclusive to ZIKV vRNA [ 32 ]. Since methylation plays an essential role in the Epstein-Barr virus (RBV) and the murine leukemia virus (MLV) [ 33 , 34 ], it will be interesting to explore the possible roles of these alterations in DENV and ZIKV.
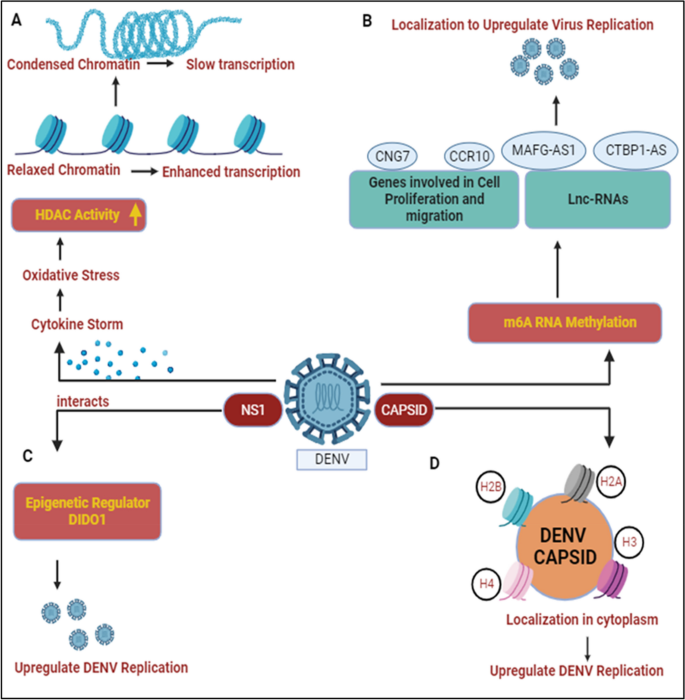
DENV and host epigenetic regulation: A DENV infection results in cytokine storm and leads to oxidative stress conditions in host cell, activating histone deacetylase (HDAC). HDAC activity condenses the chromatin and slows down the host transcription processes [ 28 ]. B DENV protein interacts with various host cellular genes/lnCRNAs and results in m6A RNA methylation. Their localization helps to enhance DENV replication [ 29 , 30 ]. C DENV NS1 interacts with DIDO1, a master epigenetic regulator and somehow elevates DENV replication [ 35 ]. D Capsid protein interacts with core histones (H2A, H2B, H3, and H4) and localizes them in cytoplasm to participate in DENV replication and propagation [ 36 ]
Dengue non-structural genes participate in enhancing virus replication by modulating various epigenetic targets. Localization studies have shown that NS1 binds with the epigenetic regulator DIDO1 (Death Inducer Obligator 1) to promote virus replication, as shown in Fig. 3 C. The silencing of DIDO1 results in reduced virus replication, indicating its significant role [ 35 ]. The dengue structural protein capsid is found to localize in the nucleus and target the four core histones, H2A, H2B, H3, and H4. DENV capsid forms heterodimers with histones and disrupts nucleosome formation for its own amplification, as shown in Fig. 3 D, Table 1 [ 36 ]. Gomes et al. provided an overview that dengue-infected patients with symptoms of haemorrhagic manifestations showed demethylation of the TNFα promoter gene and IFN-γ, leading to acute inflammation resulting in the production of a cytokine storm during dengue infection [ 59 , 60 ].
DENV and the host transcriptional machinery
Human pathogenic viruses have evolved various mechanisms to hijack host cell gene expression at the transcriptional level to facilitate their life cycle. Although the DENV genomic RNA or viral replication complexes are localized exclusively to ER-associated regions of the cytosol, structural and non-structural proteins encoded by the virus migrate to the nucleus and interact with different host proteins. Among DENV proteins, at least two, namely the C and NS5, distribute both in the cytoplasm and nucleus, getting involved in protein-protein interactions that cause the regulation of gene expression [ 61 ]. The C-protein is enriched in the nucleolus, interacting with the host protein nucleolin and facilitating viral morphogenesis [ 62 ]. Additionally, the C-protein has been indicated to mimic histone proteins and interfere with nucleosome formation, thereby influencing host cell transcription [ 36 ]. During virus infection, chemokine levels are elevated as an immune response against viruses. For instance, Interleukin-8 (IL-8) levels are upregulated in patients following infection by different group of RNA viruses such as Coronaviruses and Cytomegaloviruses to promote virus replication [ 63 , 64 ]. Similarly, In DENV-infected patients, higher IL-8 levels have been shown to correlate with severe DHF [ 65 ]. Li and co-workers showed a role for the C-protein in augmenting IL-8 transcription by associating with Positive Transcription Elongation Factor-b (P-TEFb), a complex of CDK9 and Cyclin T1 that promotes gene expression through augmentation of RNA Pol-II processivity [ 66 ]. Since pharmacological inhibition of P-TEFb showed a reversal of IL-8 upregulation, this can be a potential therapeutic strategy to avert the emergence of severe DENV, as illustrated in Fig. 4 D [ 40 , 66 ]. Although the significance of nucleolar enrichment is not clear yet, it is possible that, as observed in other RNA viruses, the DENV C-protein influences host ribosome assembly, prioritizing translation of the viral positive-strand RNA over host mRNAs [ 67 ]. Although there is no evidence that the DENV E-protein migrates to the nucleus, it has been suggested to influence host cell transcription through an interaction with TAL-1, a transcription factor involved in megakaryopoiesis, as shown in Fig. 4 A. In thrombocytopenia, only naive MEG-01 cells could be infected by DENV, and differentiated cells were resistant to virus infection/replication and it slowed the progress of megakaryopoiesis [ 37 ]. It has been observed that DENV infection enhances the activity of the Notch signaling pathway in the human system, and has been shown to limit the process of megakaryopoiesis. As, Notch-1 is known to regulate not only its downstream component RBPjk33, but also the megakaryopoiesis-specific transcription factor TAL-1. The sequestering of TAL-1 in the cytoplasm by DENV E protein may affect its availability for its transcription-related function in the nucleus, thus impeding the megakaryopoiesis. Dengue Virus also dysregulates master transcription factors involved in platelets development and maturation such as NF-E2, GATA-1, and GATA-2 and the PI3K/AKT/mTOR signaling pathway as depicted in Fig. 4 B [ 38 ].
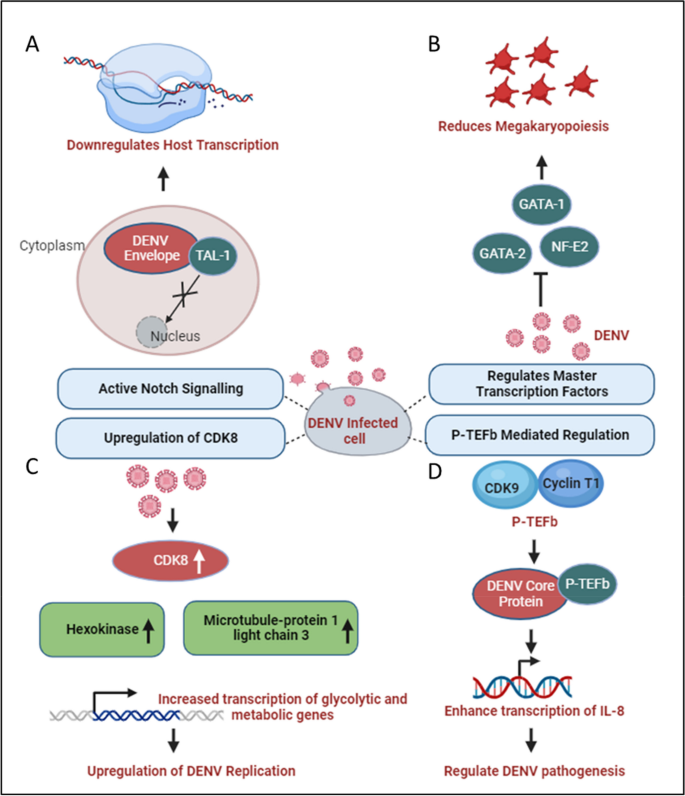
DENV and host transcriptional regulation: A DENV Envelope sequesters the transcription factor TAL-1 in the cytoplasm, affecting its transcriptional function in the nucleus [ 37 ]. B Various factors involved in transcription such as GATA-1, GATA-2, and NF-E2, which participate in the process of megakaryopoiesis, are dysregulated during dengue infection [ 38 ]. C During DENV infection, glucose and metabolic processes increase, enhancing RNA Polymerase II activity. This leads to higher expression of genes like Hexokinase and Microtubule-associated protein 1 light chain 3, promoting increased transcription of metabolic genes and enhancing DENV replication [ 39 ]. D P-TEFb interacts with DENV and activates NF-kB elements within the promoter region of IL-8 gene, enhancing IL-8 transcription which may play a significant role in DENV pathogenesis [ 66 ]
DENV exhibits distinct characteristics among its four serotypes, with DENV2, in particular, showcasing a reliance on increased glucose utilization and enhanced glucose metabolism to produce the necessary metabolic intermediates essential for viral replication [ 68 ]. During DENV-2 infection, there is an elevation in the expression of specific metabolic enzyme-encoding mRNAs, especially glucose-6-phosphate and fructose-6-phosphate in infected cells, through an upregulation in the expression of glucose transporter GLUT1 (which transports glucose from the extracellular environment into the cytoplasm) and hexokinase 2, the first rate-limiting enzyme in the glycolytic pathway [ 69 ]. DENV replication requires high glucose and metabolic intermediate for transactivation of RNA polymerase II [ 39 ]. Butler and co-workers confirmed the transcriptional upregulation of HK2 following DENV infection in a different model system and additionally showed an upregulation of Cyclin-dependent kinase 8 (CDK8) levels in DENV-infected cells to be upstream of HK2 [ 39 ]. Furthermore, the upregulation of CDK8 was shown to be beneficial for virus replication since a reversal led to a reduction in viral titers. As CDK8 expression increases, in addition to that of HK2, there is a concurrent upregulation of crucial genes like microtubule-associated protein 1 light chain 3 (LC3) and other proteins essential for virus replication. This coordinated effect is illustrated in Fig. 4 C [ 39 ].
In a study conducted by A. Carlin, the focus was on interferon-regulatory factors (IRFs), a family of transcription factors known for their role in generating type I interferon (IFN) and eliciting antiviral responses. The investigation involved triple knockout (TKO) mice, genetically engineered to lack three key transcription factors that regulate type I IFN production. Surprisingly, the TKO mice, deficient in the factors controlling type I IFN production, demonstrated increased survival rates when challenged with dengue virus (DENV). This finding strongly suggests that the antiviral effects of IRFs are mediated through interferon responses during DENV infection [ 70 ]. Contrastingly, the study also revealed a strategic countermeasure employed by the dengue virus to facilitate its replication and propagation. Dengue virus actively suppresses interferon-stimulated genes by impeding the recruitment of the transcription factor PAF1C. Additionally, the virus modulates SEC61, a key cellular component, leading to the inhibition of DENV replication. This dual nature of the host-virus interaction underscores the intricate balance between the host’s antiviral defenses, mediated by IRFs and interferon responses, and the virus’s ability to subvert these defenses for its replication advantage [ 61 ].
Depending on the model system used, DENV infection is reported to either augment or decrease the transcriptional activity of NFE2L2, encoded by the NRF2 gene. In monocyte-derived dendritic cells (moDCs), it inhibits NFE2L2 activity, whereas in differentiating megakaryocytes, it seems to augment NFE2L2 activity [ 71 , 72 ]. This manipulation is particularly prominent during instances of dengue fever (DF) and dengue hemorrhagic fever (DHF). DF is characterized by thrombocytopenia, a condition marked by a low platelet count, primarily resulting from the process of megakaryopoiesis. Despite lacking a nucleus, platelets contain essential cellular components such as cytosolic ribosomes, endoplasmic reticulum (ER), Golgi apparatus, and mitochondria, the cell’s powerhouse. These components provide the necessary machinery for viral replication and transcription [ 73 ]. In individuals infected with the dengue virus (DENV), platelets serve as a reservoir for the infectious DENV antigen. However, this aspect is controversial because other studies could not corroborate it. Consequently, severe thrombocytopenia is a prominent symptom of dengue with potential complications leading to dengue shock syndrome (DSS) in certain cases.
DENV and the host post-transcriptional machinery
Modulation of post-transcriptional gene regulation mechanisms within host cells, including alternative splicing, mRNA transport, mRNA degradation, and RNA silencing, plays a crucial role in the Dengue virus (DENV) life cycle. An understanding of these mechanisms is key to unraveling the complex interplay between the virus and its host. Among the non-structural proteins encoded by the DENV genome, NS5 and NS3 have been reported to influence host cell post-transcriptional machinery, facilitating virus replication.
In addition to its unique role in replicating viral genomic RNA (both negative and positive-sense strands) in ER-associated Replication Complexes (RC) and selectively capping positive-sense genomic RNA, NS5 localizes to the host nucleus with the aid of two Nuclear Localization Signals (NLS). This localization influences the splicing of select host cell pre-mRNAs [ 41 ]. Pre-mRNA splicing stitches gene exons together to generate unique mRNAs and is catalyzed by large RNA-protein spliceosomal complexes, termed either major (U2-dependent) or minor (U12-dependent) spliceosomes. The major spliceosome is responsible for removing 99.5% of the introns [ 74 ]. Splicing also affects mRNA transport to the cytoplasm and decoding by the translation machinery. The NS5 interactome includes core components of U5 small nuclear ribonucleoprotein (U5snRNP) complex such as CD2BP2, DDX23, etc. [ 41 ], Fig. 5 A. This modulates alternative splicing of known host antiviral factors like Cystic Fibrosis Transmembrane Regulator (CFTR), EDI (extradomain A of fibronectin), and Bclx (B-cell lymphoma-extra-large), potentially impacting the host’s immune response to Dengue infection [ 41 ]. By modulating the activities of host splicing factors, DENV can orchestrate specific splicing patterns that enhance viral gene expression and protein production [ 75 , 76 , 77 ].
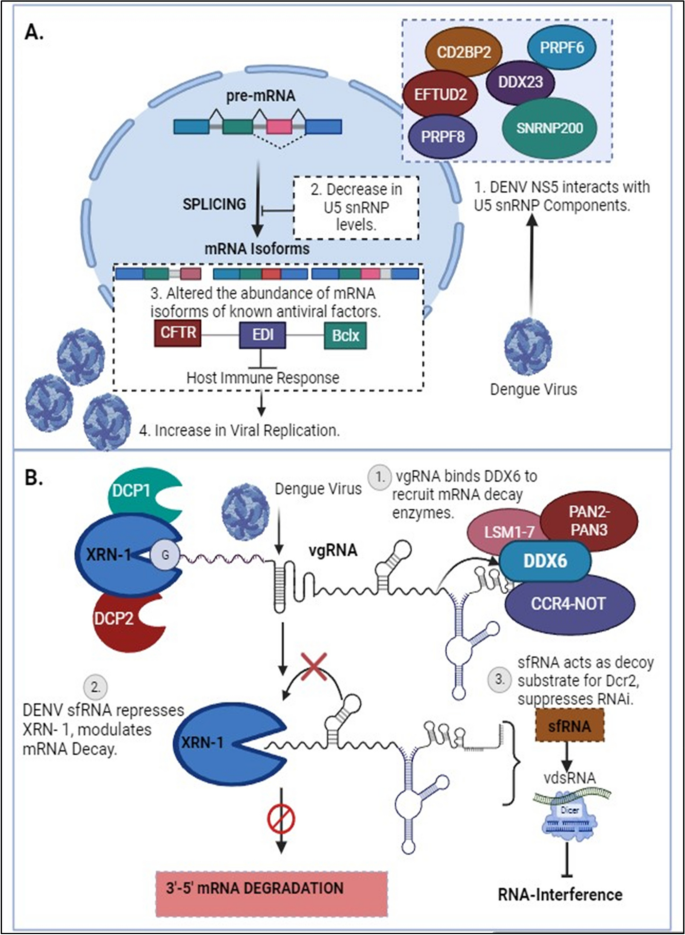
DENV and host post-transcriptional regulations: A Dengue Virus NS5 associates with active spliceosomes, interacting with key components of the U5 snRNP and sequestering them in the cytoplasm. This reduces their levels in the nucleus and alters the events of alternative splicing [ 41 ]. B In the early stages of DENV infection, viral genomic RNA (vgRNA) binds to DDX6 via the 3’UTR and recruits mRNA decay enzymes to the viral replication complex. The exoribonuclease XRN-I initiates the degradation of vgRNA, resulting in the formation of sfRNA. These pseudoknot structures play a role in stalling the XRN1 enzyme near the 5’ border of the 3’ UTR, causing the inhibition of XRN-1 and leading to modulations in mRNA degradation pathways, consequently affecting the RNAi response [ 43 ]
Polyamines (spermidine/spermine) are ubiquitous small, positively charged molecules that can function as chaperones for cellular nucleic acids [ 78 , 79 ]. Multiple viruses are known to depend on this function of polyamines for genome replication [ 80 ]. The enzyme Spermidine/spermine-N1-acetyltransferase (SAT1), an Interferon-sensitive gene (ISG), acetylates polyamines, leading to a drop in intracellular levels and thereby inhibiting viral genome replication [ 81 , 82 ]. However, SAT1 expression is downregulated upon the inclusion of exon-4 of the gene in mature mRNA, an incorporation prevented by the splicing factor RBM10 [ 81 ]. As a counter to SAT1-mediated reduction in intracellular polyamine levels, DENV NS5 interacts with RBM10, mediating its degradation by the proteasome, leading to an increase in the abundance of SAT1 mRNA containing exon-4 and a consequential reduction in SAT1 protein levels [ 42 ]. Additionally, NS5 affects the splicing efficiency of RIG-I, a sensor of viral RNA in the cytoplasm, which functions in inducing an antiviral immune response [ 41 ]. In this context, it is intriguing to note that NS5 reduces the splicing efficiency of endogenous RIG-I mRNA and also increases the expression of dominant-negative forms of IKKε (inhibitor of nuclear factor kappa-B kinase ε) during DENV infection. This, by all means, results in supporting pro-viral conditions in the cell. Hence, it can be appropriately proposed that the interaction of NS5 with the splicing machinery is an immune suppression strategy [ 41 ]. Extending these studies to include other members of the flavivirus family, a study by Michalski et al. suggested that ZIKV sfRNAs also affect host mRNA splicing. ZIKV sfRNAs can sequester splicing factors like PHAX, PPIH, SF3B1/2, and NMP1, which are involved in regulating alternative splicing of host transcripts, significantly contributing to the mis-regulation of cellular RNA splicing, including exon-skipping and intron retention events in various genes during infection [ 83 ].
In response to virus infection, host cells secrete interferons, which bind to cognate receptors on the cell surface and activate the expression of IFN-sensitive genes through signal transduction. STAT2 forms an essential component of this signal transduction pathway, and suppression of this protein leads to a suppression of the innate host antiviral response. While in the cytosol, NS5 associates with the host STAT2 protein, catalyzing its degradation and the consequent silencing of STAT2-dependent gene expression, in the nucleus, NS5 interacts with a multiprotein complex involved in STAT2-independent gene expression and negatively regulates its function [ 53 ]. Petit and coworkers showed that through interaction with PAF1, a component of the Polymerase Associated Factor 1 complex (PAF1C), which is instrumental in the expression of STAT2-independent immune response genes, DENV NS5 inhibits its function, leading to suppression of the antiviral genes targeted by PAF1C [ 84 ].
During dengue infection, the virus interacts with the host cellular machinery responsible for 5’ capping. DENV employs multiple strategies to hijack and manipulate this process. For instance, the virus sequesters essential host factors involved in capping, such as the cap-binding complex and associated enzymes. By doing so, DENV ensures efficient capping of its own viral transcripts, enhancing their stability and translational competence [ 85 ]. During dengue infection, the antibodies produced are generally cross-reactive among DENV serotypes, carrying a higher risk of promoting Antibody-Dependent Enhancement (ADE) [ 86 ]. A study by Narayan and Tripathi, demonstrated that dengue antibody-dependent enhancement (ADE)-mediated virus entry predominantly causes the enrichment of differentially expressed genes (DEGs) that regulate RNA processing. Many of these factors are known to interact with dengue viral RNA, suggesting that dengue ADE modulates the transcriptome of monocytes to upregulate genes responsible for vesicular transport and mRNA processing [ 87 ]. Additionally, the spatial regulation of mRNA transport during dengue infection is mediated by the interaction between viral proteins and host factors involved in mRNA transport processes. For example, RNA-binding proteins (RBPs) are key regulators of mRNA transport and localization. HnRNPs (heterogeneous nuclear ribonucleoproteins), for instance, have been shown to regulate host mRNA expression in response to dengue infection. Modulation of these RBPs by dengue infection can impact mRNA transport and localization, potentially influencing viral replication and pathogenesis. Furthermore, mRNA transport in Dengue infection can influence the host immune response. Viral RNA molecules transported to specific subcellular compartments may interact with immune sensors, such as pattern recognition receptors (PRRs), triggering antiviral immune responses. In addition, the cytosolic localization of viral mRNA molecules can impact the accessibility of viral RNA to host immune factors, influencing the recognition and clearance of the virus [ 88 ].
One pivotal facet of post-transcriptional regulation is mRNA turnover, which involves the mRNA decay machinery to synchronize gene expression by balancing the stability and lifespan of mRNAs through regulated mechanisms. Efficiently evolving viruses exploit the cellular gene expression machinery to escape antiviral responses and control viral replication [ 89 ]. Viral genomic material, in addition to virus-encoded factors and enzymes, interacts with elements of the host mRNA decay pathways to modulate the half-life of both viral and cellular mRNAs. Furthermore, viruses encode ribonucleases to degrade cellular mRNAs, providing multiple advantages, one of which is increased stability of viral transcripts. Additionally, it has been demonstrated that the dengue virus regulates XRN1 (5’-3’ exoribonuclease 1 protein), a key component of the cellular mRNA decay machinery responsible for degrading mRNAs in the 5’ to 3’ direction. DENV undertakes this modulation through subgenomic flavivirus RNA (sfRNA), a non-coding RNA molecule derived from the 3’ untranslated region (UTR) of the viral genome. This sfRNA can bind directly to XRN1, inhibiting its exonuclease activity. By inhibiting XRN1, sfRNA prevents the degradation of viral RNA, including non-coding regions, allowing the virus to maintain a stable pool of genomic RNA for replication. Additionally, by inhibiting XRN1, the virus stabilizes host mRNA, hindering the process of mRNA degradation and potentially dampening the host immune response, as shown in Fig. 5 B [ 43 ]. This particular mechanism of stalling and repression of XRN1 by generating sfRNA appears to be a common strategy by many members of Flavivirus family. Zika virus targets the cytoplasmic 5’-3’-exoribonuclease XRN1, by interacting with the cytoplasmic RNA decay machinery, which results in the suppression of gene expression and the generation of copious amounts of sfRNA in infected cells. Notably, XRN1 targeting is not limited to the Flaviviruses but is employed by RNA viruses from diverse evolutionary backgrounds as part of the molecular arms race to help the infecting virus usurp the innate immune response of the host. Further studies, elucidate that DENV-2 sfRNAs interact with and sequester two viral restriction factors involved in aspects of cellular RNA decay, DDX6 and EDC3, in addition to a deadenylase component CNOT1. Similar results have been obtained with the ZIKIV sfRNA, demonstrating that besides the RNA decay pathway, these viruses also reduce the decapping and deadenylation of transcripts [ 83 ].
During dengue infection, several mRNA degradation pathways have been implicated, including exonucleolytic degradation mediated by the exosome complex, endonucleolytic cleavage by endoribonucleases, and the miRNA-mediated decay pathway [ 90 ]. To aid viruses with their establishment inside the host, these viruses encode several proteins that interact with various host factors. One such class of proteins identified as Viral Suppressors of RNA Silencing (VSR) has been demonstrated to interact with components of the host machinery participating in post-transcriptional gene silencing, a known antiviral defense mechanism. Based on the present study, it has been shown that dengue NS3, a known VSR, interacts with a cellular chaperone, Heat shock protein family A (Hsp70) member 1A (HSPA1A), subsequently modulating its expression levels as well [ 91 ]. Moreover, it was discovered that HSPA1A is linked with the host RNA silencing machinery by coordinating with the Argonaut proteins, Ago1, and Ago2, and translocating to the cytoplasm of the cell along with them. Hence, these outcomes provide evidence for the participation of other host partners in mediating the VSR role of dengue NS3 and in determining the mechanisms of RNA silencing in the case of Dengue virus infection. Furthermore, one of the key mediators of antiviral immunity is Interferon-stimulated genes (ISGs) that play a crucial role in the immune response to DENV infection. IRAV is an RNA-binding protein, which functions as an ISG and localizes to cytoplasmic processing bodies (P bodies) in uninfected cells, where it interacts with the MOV10-RISC complex RNA helicase (MOV10) and Upstream frameshift 1 (UPF1), a key component of nonsense-mediated RNA decay pathways. IRAV and MOV10 are believed to function in conjunction with other proteins like HuR, LARP1, and PABPC (some RNA-binding proteins involved in the mRNA decay pathway) to operate in the disruption of viral RNA by colocalizing with the DENV replicating complex, particularly NS3 and NS4A, thus possessing anti-viral activity. A study by Balinsky et al. in 2017 proposed that IRAV is upregulated during DENV infection [ 44 ].
DENV and the host translational machinery
The Dengue virus, functioning as an intracellular parasite, has developed sophisticated strategies to manipulate the host’s translational machinery, ensuring efficient viral protein synthesis and replication. This intricate interplay between the virus and the host’s translation machinery is crucial for the successful propagation of the virus within the host cell. Upon infection, the Dengue virus genome, a positive-sense single-stranded RNA, serves as a template for the translation of viral proteins. Translation initiation primarily occurs through the cap-dependent translation mechanism [ 92 ]. In this process, the cap-binding protein complex (CBC), specifically the eIF4E factor, recognizes the cap structure at the 5’ end of the viral RNA. Subsequently, the eIF4F complex, comprising eIF4E, the adapter protein eIF4G, and the helicase eIF4A (along with its cofactor eIF4B), binds to the cap and recruits ribosomes to the viral mRNA, facilitating translation initiation [ 45 , 93 ].
In addition to the aforementioned mechanisms, Bidet and Garcia-Blanco identified the DENV-2 NS3 protein as an interacting partner of various translation initiation factors, namely eIF4G, eIF5A, and eIF3L, showcasing the importance of these proteins in upregulating viral RNA translation. This observation underscores DENV-2’s ability to target key components of the host’s translation initiation machinery [ 94 ]. Furthermore, these findings extend beyond DENV; the NS5 protein of the Yellow Fever virus (YFV) was also found to interact with these translation factors in two-hybrid screening assays [ 95 ]. Translation elongation factors, such as eEF1A and eEF2, have also been shown to be essential for WNV and Yellow Fever Virus 17D (YFV17D), respectively, confirming the importance of these protein categories in flaviviral infections, besides the capacity of flaviviruses to manipulate the host’s translational apparatus [ 96 ].
The availability of eIF4E is a key control point for translation. Hypophosphorylation of eIF4E-binding proteins (4E-BPs) prevents their interaction with eIF4E in the cap-binding complex, resulting in the suppression of cap-dependent translation. In such situations, an internal ribosome entry site (IRES) is utilized to initiate cap-independent translation. IRES-mediated translation involves unique sequences present in the Dengue virus 5’-untranslated region (UTR), which recruit ribosomes directly [ 97 , 98 ]. A proposed non-canonical model suggests that during low levels of eIF4E, RNA sequences in the 3’ UTR stabilize translation initiation factors at the 5’ end, subsequently recruiting eIF4G and eIF4A, thereby bypassing the requirement for eIF4E. This process facilitates the formation of a bridge between the 5’ UTR and the 3’ UTR, enhancing translation under these conditions [ 98 ]. A similar phenomenon is observed in other genera within the Flaviviridae family; hepatitis C virus (HCV) contains an IRES to facilitate translation initiation. HCV recognizes the 43S particle primarily through IRES RNA interaction. This direct interaction helps in initiating translation processes [ 99 ]. Interestingly, Polypyrimidine tract-binding protein (PTBP) protein helps other viruses, such coxsackievirus B3 (CVB3), circularise the viral RNA by bridging the UTRs necessary for effective translation of viral RNA [ 100 ].
The Dengue virus 3’ UTR stimulates translation through both cap-dependent and cap-independent mechanisms. Furthermore, the 3’ stem-loop structure complements this process by enhancing polysome formation. While the lack of a 3’ poly(A) tail in the Dengue virus genome is not entirely clear, the 3’ stem-loop domain is functionally analogous to a 3’ poly(A) tail, as it recruits translation initiation factors [ 97 ]. Notably, the 3’ stem-loop functions at both the translation and replication levels, as the Dengue virus RNA serves as both mRNA and a template for negative-strand synthesis [ 101 ]. In contrast CVB3 contains hexa-nucleotide stretch within stem loop C, which is critical for CVB3 IRES mediated translation [ 102 ]. Multifunctional RNA-binding proteins (RBPs) such as G3BP1 (G3BP Stress Granule Assembly Factor 1), G3BP2 (G3BP Stress Granule Assembly Factor 2), and Caprin1 are identified as novel regulators during Dengue virus type 2 (DENV-2) infection. They play a significant role in interferon-stimulated genes (ISGs) translation. It has been reported that DENV-2 sub-genomic flaviviral RNA binds to G3BP1, G3BP2, and Caprin1, leading to the inhibition of ISG mRNA translation, resulting in a proviral effect [ 46 ].
A notable phenomenon observed in Dengue virus translation is the binding of the poly (A)-binding protein (PABP) to the non-polyadenylated 3’ untranslated region (3’ UTR) of the viral RNA. Specifically, PABP interacts internally with the 3’ UTR, positioning itself upstream of the conserved 3’ stem-loop structure near the two dumbbell structures. The PABP-interacting protein 2 (PAIP2), a translation inhibitor specific to PABP, plays a role in interfering with the interaction between the Dengue virus 3’ UTR and PABP. In-vitro experiments using baby hamster kidney cell extracts revealed that PAIP2 inhibits the translation of Dengue virus reporter RNAs in a dose-dependent manner, underscoring its impact on the translational process. These findings shed light on the broader understanding of PABP’s translation mechanism, demonstrating its ability to bind to viral RNA even in the absence of a terminal poly(A) tail. This insight illuminates the intricate regulatory mechanisms governing the translation of Dengue virus RNA and provides potential avenues for therapeutic interventions [ 47 ].
DENV and the host post-translational machinery
The genome of the Dengue virus initiates the formation of a single polypeptide through translation, subsequently processed to yield various functional and non-functional proteins. These proteins undergo post-translational modifications (PTMs), as depicted in Fig. 6 . Given DENV’s lack of its enzymatic machinery for PTMs, it relies on the host’s PTM system for survival [ 103 ]. These modifications involve the covalent addition of small protein molecules or functional groups to specific amino acid residues, including ubiquitination (addition of ubiquitin), lipidification (addition of lipid), glycosylation (addition of carbohydrate), and chemical group modifications such as hydroxylation, phosphorylation, acetylation, and methylation [ 49 ].
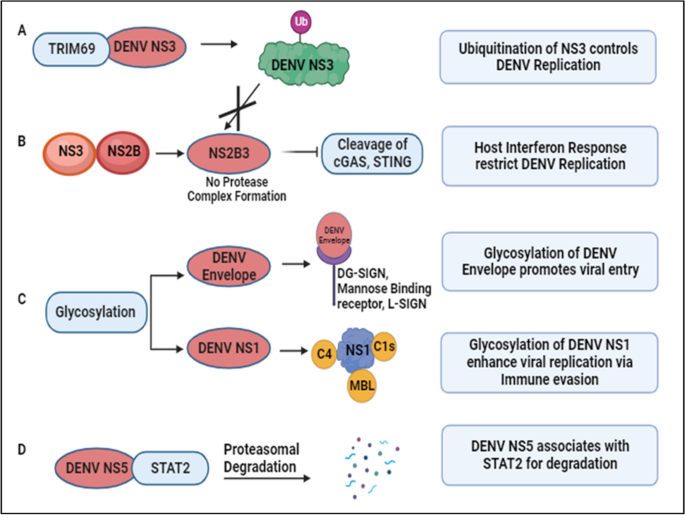
DENV and host post-translational regulation: A DENV NS3 interacts with TRIM69, induced as an Interferon Stimulated Gene (ISG), activating the ubiquitination pathway to restrict DENV replication [ 48 ]. B Conversely, ubiquitination of NS3 prevents the formation of the protease complex (NS2B3), inhibiting the cleavage of Interferon response-related genes, namely cGAS and STING. This results in an elevated host interferon response, targeting DENV replication [ 104 , 105 ]. C Glycosylation of DENV proteins aids attachment to host surface receptors and various complementary proteins, contributing to immune evasion and viral propagation [ 49 , 50 , 51 , 52 ]. D DENV NS5 interacts with STAT2, preventing its phosphorylation and targeting it for proteasomal degradation [ 53 , 106 ]
During infection, these modifications can exert either proviral or antiviral effects by activating or inhibiting proteins or modulating the immune response. An example is the TRIM family protein TRIM69, an interferon-stimulated gene (ISG). TRIM family members, pivotal in the innate immune pathway, play diverse roles from transcription to post-translation. During DENV infection, TRIM69 interacts with DENV NS3, activating the ubiquitination pathway and restricting DENV replication, as illustrated in Fig. 6 A [ 48 ]. Ubiquitination involves covalently attaching ubiquitin to the C-terminal glycine residue of the target protein. DENV NS3, in association with NS2B, forms a protease complex (NS2B3) that cleaves cyclic GMP-AMP synthase (cGAS) and Stimulator of Interferon Genes (STING). Ubiquitination of DENV NS3 prevents its proteolytic degradation, enhancing viral replication. However, reduced degradation of STING and cGAS enhances the interferon response, acting as host defense mechanisms against DENV as noted in Fig. 6 B [ 104 , 105 ]. To target the host cell, DENV utilizes the host’s glycosylation machinery for glycan conjugation on its proteins. Glycosylation, involving attaching glycan moieties to serine or threonine amino acid residues, plays critical roles in DENV entry, assembly, virulence, and pathogenicity. Receptors such as DC-SIGN, mannose-binding receptors, and L-SIGN receptors facilitate DENV entry by recognizing glycans on envelope proteins as shown in Fig. 6 C [ 49 , 50 , 51 ]. Glycosylated DENV NS1 contributes to protein secretion and stability, binding to complement components and preventing lectin complement activation and DENV neutralization, regulating DENV pathogenesis [ 52 ].
Studies reveal novel mechanisms of immune evasion and potential antiviral therapeutic targets. DENV NS5 associates with Signal Transducer and Activator of Transcription 2 (STAT2), a critical component in interferon signaling, preventing its phosphorylation and targeting it for proteasomal degradation, as depicted in Fig. 6 D [ 53 , 106 ]. Additionally, STAT3 upregulation and activation negatively regulate Type I and Type III interferon responses during DENV-2 infection, suggesting STAT3 as a proviral factor for DENV propagation and a potential antiviral target [ 54 ]. Through immunoprecipitation coupled with mass spectrometry analysis, Zhang et al. identified host proteins exhibiting differential ubiquitylation patterns in DENV-infected cells. Notably, they found that the lipid droplet-localized type-III membrane protein AUP1 undergoes deubiquitylation upon DENV infection. This virus-induced deubiquitylation leads to increased AUP1 levels and subsequent accumulation within autophagosomes. Importantly, AUP1 interacts with DENV NS4A protein, promoting viral production. Interestingly, a ubiquitin-modified mutant of AUP1 suppressed the interaction with NS4A, resulting in impaired lipophagy and defective viral replication. These findings implicate ubiquitination as a critical host regulatory mechanism that DENV modulates to facilitate its replication cycle. Further studies are needed to fully elucidate the ubiquitylation changes induced by flaviviral infection [ 107 ].
In a study employing MS-based phosphoproteomics, the global host phosphorylation profile was investigated for DENV [ 108 ], WNV [ 109 ], and ZIKV, revealing shared cellular pathways whose phosphorylation status alters across different flaviviral infections, affecting various RNA processing and metabolic pathways [ 110 ]. Using the same strategy, the authors identified 604 host proteins differentially phosphorylated in JEV infected human U251 glial cells. Importantly, pharmacological inhibition of the JNK1 pathway in mice infected with JEV resulted in reduced inflammatory cytokine secretion, lower viral load, and increased survival rates. This indicates that the JNK1 signaling pathway, modulated by JEV-induced phosphorylation events, plays a critical role in driving the inflammatory response and pathogenesis during JEV infection [ 108 ].
DENV and the host stress responses
DENV relies on host cellular processes for both its replication and translation. Upon infection, DENV intricately interacts with the host’s immune system, triggering a cascade of cellular and molecular responses aimed at combating the invading pathogen. Among these responses, host stress responses play a crucial role in modulating the outcome of DENV infection. Stress Granule (SG) formation is one of the consequences of cellular stress, heat shock, dysregulation of molecular mechanisms, and certain viral infections. Alterations in protein expression mechanisms results in SG assembly [ 111 ].
Molecules such as T cell intracellular antigen (TIA1), TIA-1 related protein (TIAR), and Ras-Gap-SH3 domain-binding protein (G3BP) are among the biomarkers of SG, regulated during cellular stress [ 112 , 113 ]. TIA1 and TIAR interact with DENV NS3, and colocalize in the perinuclear regions to enhance viral RNA formation and prevent SG formation, as shown in Fig. 7 A. In contrast TIA1 and TIAR interacts with WNV 3’ (-) SL RNA to facilitate the virus replication [ 55 ]. Additionally, one study reported that DENV UTRs interact with host cellular proteins such as DDX6, G3BP1, G3BP2, Caprin-1, and USP10 as noted in Table 1 [ 56 ]. These proteins play roles in SG formation, but during DENV infection, they colocalize with DENV RNA, specifically interacting with DENV 3’UTR, significantly influencing DENV replication [ 56 ]. In contrast, G3BP1 and Caprin-1 form a stable complex with the capsid protein of ZIKV to benefit virus replication [ 114 ]. Furthermore, the capsid protein of JEV inhibits (arsenite-induced) SG formation by interacting with the SG protein Caprin-1 [ 115 ]. DENV employs various strategies to hinder SG assembly to enhance its survival. Valosin-containing protein (VCP), a cellular ATPase, reportedly plays a crucial role in DENV replication. VCP, together with Nuclear Protein Localization 4 (NPL4), forms a complex to enhance DENV propagation and disassembles stress granule formation. DENV NS4B activates VCP to evade stress response, preventing SG formation, while simultaneously facilitating cellular translation to enhance DENV replication and translation [ 57 ].
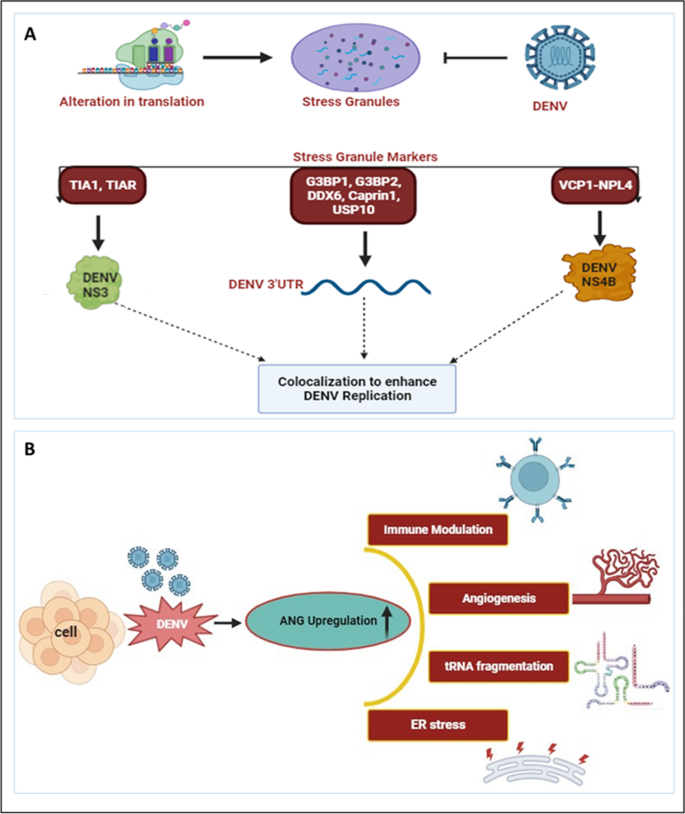
DENV and host stress responses: A Alterations in translation processes result in the assembly of stress granules. However, DENV prevents stress granule assembly by interacting with various stress granule markers. TIA1 and TIAR interact with DENV NS3, while various proteins such as G3BP1, G3BP2, DDX6, Caprin1, and USP10 bind to DENV 3’UTR. Additionally, VCP1, together with NPL4, forms a complex with DENV NS4B. These interactomes colocalize and participate in DENV replication [ 55 , 56 , 57 ]. B During DENV infection, Angiogenin levels have been found to be enhanced compared to uninfected controls. The upregulated Angiogenin may play a crucial role in various processes, including immune modulation, angiogenesis, tRNA fragmentation, and ER stress, among others [ 116 ]
Several studies have indicated the modulation of RNases upon DENV infection. The activity of various ribonucleases (RNases) such as DICER, ELAC2, Angiogenin produced during host stress conditions, including virus infection, results in the formation of various regulatory RNAs [ 117 ]. Recently we have reported the synergistic correlation between host ribonuclease Angiogenin expression and DENV replication. Angiogenin (also known as RNase 5) is a member of the Ribonuclease A superfamily. Our finding revealed that, angiogenin levels are elevated during DENV infection. Interestingly, angiogenin levels remain unaffected by siRNA knockdown, suggesting that dengue infection may directly regulate angiogenin expression. As illustrated in Fig. 7 B [ 116 ], upregulation of angiogenin during DENV infection may have a possible proviral effect, contributing to the replication and propagation of the dengue virus.
Dengue virus infection is known to induce endoplasmic reticulum (ER) stress, triggering signaling pathways associated with ER stress during viral infections. These pathways activate genes that support cell survival and anti-viral defenses. Viruses exploit the host’s cellular machinery, particularly the ER, to produce mature viral offspring and facilitate their replication. In response to the stress induced by alterations in protein production, the ER activates the Unfolded Protein Response (UPR) pathway to maintain ER homeostasis. Upon DENV infection, various UPR elements such as PERK, ATF6, and IRE1α are activated, which in turn activate innate immune factors, such as IRF3, PKR, and NF-κB. This approach might be employed to reduce viral infection. Thus, UPR components could serve as potential target of therapy for reducing DENV replication [ 118 , 119 ].
DENV-NS1 alters cellular behaviour to increase the secretion of extracellular vesicles (EVs) containing miR-148a. These EVs are internalized by human microglial cells, where miR-148a interacts with the 3’UTR region of the ubiquitin-specific peptidase 33 (USP33) protein, leading to its downregulation. Consequently, reduced USP33 expression results in decreased levels of the activating transcription factor 3 (ATF3) protein. ATF3 plays a crucial role in regulating genes involved in proinflammatory pathways, such as TNF-α, NF-κB, and IFN-β. This study elucidates how DENV exploits the EV pathway to deliver miR-148a, thereby modulating USP33 and ATF3 levels in human microglial cells, ultimately contributing to inflammation within the Central Nervous System (CNS) [ 58 ].
DENV and antiviral therapeutics
While there is currently no specific antiviral strategy approved to treat the dengue infection, ongoing research has explored various approaches to combat the infection. These strategies include investigating compounds with the potential to inhibit viral replication, immune modulators intended for enhancing the host’s immune response, and monoclonal antibodies designed to mimic the body’s natural defenses. Additionally, vaccine development has been a focus not only for prevention but also for potential therapeutic interventions. Several candidates are currently undergoing clinical trials as part of significant global efforts toward developing a preventive dengue vaccine. One of these, known as Dengvaxia, has been implemented and licensed in humans in several dengue-endemic countries. However, this vaccine has limitations, including approximately 60% efficacy and an association with antibody-dependent enhancement of disease severity in children, which impede its utilization. As of today, due to certain challenges, drug discovery efforts have not been able to provide any approved therapeutics to treat dengue virus infection [ 120 ].
Recently, Obi JO et al. described a novel small molecule inhibitor, JNJ-1802. JNJ-1802 target the DENV NS4B protein and hinders the NS3 and NS4B interplay, as shown in Fig. 8 A [ 121 ]. JNJ-1802 has demonstrated an effective barrier to resistance, in-vitro antiviral activity at low concentrations, and robust in-vivo efficacy in mice against four DENV serotypes. It has completed a phase I first-in-human clinical study in healthy individuals and was found to be safe [ 122 ]. Additionally, they also reported a compound, JNJ-A07, that exhibits antiviral activity against 21 clinical isolates of the dengue virus, spanning all known serotypes and genotypes of the dengue virus with a similar mechanism of action, but JNJ-1802 was selected over JNJ-A07 due to better preclinical safety profile [ 123 ]. Furthermore, several other DENV NS4B inhibitors have been developed, showing effectiveness as antivirals against dengue virus infection. Xie et al. demonstrated antiviral activity of NITD 618, with EC 50 values ranging from 1.0 to 4.1 µM. This compound functions by inhibiting RNA synthesis, but its poor pharmacodynamics make it challenging for further development as a drug against DENV infections [ 124 ]. Similarly, SDM25N, an opioid receptor antagonist, was identified, and its activity also relies on NS4B, restricting RNA genome replication [ 125 ]. Another group also identified a similar compound, AM404, whose interactions with NS4B are yet to be validated [ 126 ]. Additionally, a spiropyrazolopyridone compound was detected that likely binds to valine 63 (V63) residue of NS4B present in DENV-2 and DENV-3, and restricts virus replication in the stated serotypes [ 127 ]. Hence, this candidate known as compound-14a was considered a potential preclinical contender for future development. Similarly, phenotypic screening of a Novartis compound library with 1.5 million compounds led to the identification of 13,000 potential inhibitors, selecting a hit, NITD-688, that displayed EC 50 values of 8–38 nM inhibiting all DENV serotypes [ 128 ]. The compound’s interaction with NS4B was thoroughly characterized by NMR studies, demonstrating its potential for use in clinical research. Overall, these compounds validate DENV NS4B a validated target for the development of antivirals. It has been established that compounds or inhibitors that target DENV non-structural proteins interfere with viral replication and are possible targets for the development of antiviral treatments. Several drugs, namely Peptide 3, Peptide 4, Peptide 10, and Peptide 11, are involved in the inhibition of the NS1 protein as noted in Table 2 [ 129 ].
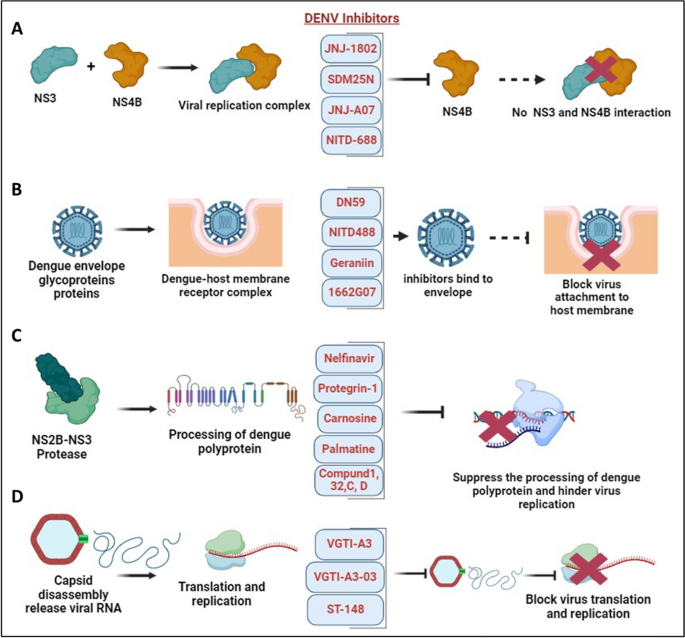
DENV and antiviral therapeutics: A Interaction between DENV NS3-NS4B in the formation of the virus replication complex. Several novel small molecule inhibitors, including JNJ-1802, JNJ-A07, SDM25N, and NITD-688, have been identified to inhibit NS4B, disrupting its interaction with NS3 and thereby suppressing DENV replication [ 122 , 125 , 128 ]. B DENV fusion inhibitors such as Geraniin, DN59, NITD-488, and 1662G07 bind with envelope protein thereby prohibiting virus attachment and entry into the host membrane [ 130 , 131 , 132 , 133 ]. C NS2B-NS3 protease complex participate in the processing of dengue polyprotein and supports virus replication. Inhibitors such as Nelfinavir, Protegrin-1, Carnosine, Palmatine, Compund 1, 32, C, D targets NS2B-NS3 protease complex and hinder virus replication [ 134 , 135 , 136 , 137 ]. D Dengue capsid protein undergoes capsid disassembly, releasing viral RNA for translation and replication. Inhibitors such as VGTI-A3, VGTI-A3-03, and ST-148 interact with the capsid protein, inducing antiviral effects and hindering dengue virus translation and replication [ 138 , 139 ]
In the pursuit of effective therapeutics against DENV, researchers have targeted specific viral proteins crucial for its replication within host cells. Among these targets, the NS3 helicase and NS2B-NS3 protease have garnered considerable attention due to their essential roles in viral replication. Ivermectin, a drug well known for its antiparasitic properties, has emerged as a promising candidate for DENV treatment. Suputtamongkol et al. [ 140 ] reported on a phase 2/3 clinical trial investigating Ivermectin’s efficacy, revealing its ability to suppress NS3 helicase and NS2B-NS3 protease activity, consequently hindering DENV replication in host cells as mentioned in Table 3 . Various compounds have been explored for their potential to inhibit NS3-helicase and NS2B-NS3 protease activity, thereby disrupting viral replication. Lee et al. [ 137 ] outlined several promising candidates, including ST-610 [ 141 ] and Suramin [ 142 ] as NS3-helicase inhibitors, alongside a range of compounds targeting NS2B-NS3 protease such as Protegrin-1 [ 134 ], Retrocyclin-1 [ 135 ], Nelfinavir, Carnosine, Palmatine, and Thiazolidinone-peptide hybrids. Additionally, compounds like Compound 32, Compound 1, 166347, ARDP0006, ARDP0009, Compound 7n, Diaryl(thio)ethers, Compound C, and Compound D have shown potential in inhibiting NS2B-NS3 protease activity [ 136 , 137 ].
In addition to this, several drugs are designed to target structural proteins, as described in Table 2 . For instance, 1662G07 and some analogs target the envelope protein as DENV fusion inhibitors [ 130 ]. Likewise, DN59 [ 131 ], and NITD448 [ 132 ] similarly inhibit the E-protein. Compound like Geraniin target the E-protein to inhibit virus binding and entry as shown in Fig. 8 B [ 133 ]. Furthermore, MLH40 inhibits the interplay between the envelope and the membrane proteins by specifically targeting the prM/M protein [ 143 ]. VGTI-A3 and VGTI-A3-03, by binding with the capsid protein, hinder the interplay between the DENV C protein and host intracellular lipid droplet, thereby disrupting the binding and thus prohibiting virus entry into cells [ 138 ]. A study by Xia et al. demonstrated the effect of a novel compound, ST-148, that inhibits the capsid protein of the dengue virus and interferes in virus replication as mentioned in Fig. 8 D. ST-148 interacts with the α1-α1′ helices region of the capsid protein, resulting in reduced viremia as well as low cytokine levels in the plasma of DENV-infected AG129 mouse model. One structural approach showed that ST-148 promotes two capsid dimers to engage in a “kissing” interaction [ 139 ]. However, a recent investigation reported that ST-148 inhibits DENV-2 but not DENV-1, DENV-3, and DENV-4. The antiviral and resistance mechanisms of ST-148 are yet unclear [ 148 ]. Pre-clinical data of some antiviral agents in the advance stage have shown potential effect against DENV. AT-752, an inhibitor of NS5 RdRp function, is in Phase 2 clinical trial as mentioned in Table 3 [ 145 ]. It reduced the viremia in immunocompromised mouse models infected with DENV. Ketotifen, a drug in Phase 4 clinical trial, reduced vascular leakage in a mouse model infected with DENV [ 147 ]. Zanamivir, and UV-4B as mentioned in Table 3 are still in the early phase of clinical trial [ 144 , 146 ]. Although many of these molecules and compounds are promising candidates for further development, significant limitations of these antivirals involve cytotoxicity and adverse effects. Moreover, clinical trials are crucial for evaluating the safety and efficacy of these antiviral therapies, and their success holds promise for advancing the treatment options available for dengue patients.
The root cause and progression of the disease are significantly influenced by the complex molecular interactions between the dengue virus and the host cellular machinery. In this review, we delve into the interplay between DENV and the host molecular machinery, focusing on epigenetic regulations, transcription, post-transcription, translation, and post-translation processes, including stress granules. These factors play a crucial role in shaping the evolution of an infection, determining the severity of symptoms, and influencing the host body’s defense system response. Epigenetic alterations such as DNA methylation, histone modifications, and involvement of various non-coding RNAs regulate DENV replication, immunological responses, and disease development. The virus exploits these alterations, manipulating host cellular components to facilitate its proliferation and evade immune responses. Transcriptional regulation is another essential aspect of dengue infection, as the virus manipulates the host’s transcriptional machinery to support its development and hinder immune responses. By appropriating key transcription factors and regulators, DENV modifies gene expression patterns, impacting both immune responses and viral pathogenesis.
Similarly, DENV exerts control over its own gene expression and alters the host cell environment through modulating post-transcriptional modifications, including alternative splicing and RNA degradation. Furthermore, at the translational level, DENV competes with host translation factors, employing techniques such as internal ribosome entry sites (IRES) to initiate translation and ensure efficient protein synthesis. Post-translational changes of both DENV and host proteins also play a role in viral replication, immune evasion, and host response regulation. Understanding these complex interactions at the molecular level is imperative for developing efficient diagnostic, therapeutic, and preventive measures against dengue virus infection. Targeting specific elements within these regulatory networks may open new avenues for therapeutic development and intervention, ultimately reducing the impact of this pervasive and often severe dengue infection.
Availability of data and materials
Not applicable.
Abbreviations
Dengue fever
Dengue hemorrhagic fever
Dengue shock syndrome
Non-steroidal anti-inflammatory medicines
Antibody-dependent enhancement
Antibody-dependent cellular cytotoxicity
Complement system
Untranslated Region
Cyclin dependent kinase 8
Hexokinase 2
C-C Motif Chemokine Receptor 10
C-terminal-binding protein antisense 1
MAF BZIP transcription factor G antisense RNA 1
Interferon gamma
Tumour necrosis factor α
CCCTC‐binding factor
Positive transcription elongation factor b
Nuclear factor kappa B
Interleukin-8
T-Cell Acute Lymphocytic Leukemia 1
Small nuclear ribonucleoproteins
CD2 cytoplasmic tail binding protein 2
DEAD box helicase 23
5’-3’ Exoribonuclease 1 protein
Retinoic acid-inducible gene I
Inhibitor of nuclear factor kappa-B kinase ε
Differentially expressed genes (DEGs)
RNA-binding proteins
Heterogeneous nuclear ribonucleoproteins
Sub genomic flavivirus RNA
Interferon-stimulated genes
Viral Suppressors of RNA Silencing
Heat shock protein family A (Hsp70) member 1A
MOV10-RISC complex RNA helicase
Upstream frameshift 1
Viral genomic RNA
RNA Interference
Eukaryotic translation initiation factor 4E
G3BP Stress Granule Assembly Factor 1
G3BP Stress Granule Assembly Factor 2
Cap binding complex
Internal ribosomal entry site
- Post-translational modifications
Tripartite motif family members
Cyclic GMP-AMP synthase
Stimulator of interferon genes
Dendritic cell specific ICAM3 grabbing non-integrin receptor
Mannose binding lectin
Signal transducer and activator of transcription 2
Histone deacetylase
DNA methyltransferase
Death inducer obligator 1
Stress granules
T cell intracellular antigen
TIA-1 related protein
Ras-Gap SH3 domain binding protein
Ubiquitin Specific Peptidase 10
Valosin containing protein
Nuclear protein localization 4
Extradomain A of fibronectin
B-cell lymphoma-extra-large
Cystic fibrosis transmembrane regulator
RNA-binding protein 10
Hu Antigen R
La- Related Protein 1
Cytoplasmic poly(A)-binding protein
Poly(A) Binding Protein Interacting Protein 2
PKR-like ER kinase
Activating transcription factor 6
Activating transcription factor 3
Inositol-requiring enzyme 1 alpha
Protein kinase R
Interferon regulatory transcription factor 3
Ubiquitin carboxyl-terminal hydrolase 33
Guzman MG, Gubler DJ, Izquierdo A, Martinez E, Halstead SB. Dengue infection. Nat Rev Dis Prim. 2016;2:1–26. https://doi.org/10.1038/nrdp.2016.55 .
Article Google Scholar
Tuiskunen Bäck A, Lundkvist Å. Dengue viruses – an overview. Infect Ecol Epidemiol. 2013;3(1):19839.
Google Scholar
Perera R, Kuhn RJ. Structural proteomics of dengue virus. Curr Opin Microbiol. 2008;11(4):369–77.
Article CAS PubMed PubMed Central Google Scholar
Byk LA, Gamarnik AV. Properties and functions of the dengue virus capsid protein. Annu Rev Virol. 2016;3:263–81.
Libraty DH, Young PR, Pickering D, Endy TP, Kalayanarooj S, Green S, et al. High circulating levels of the dengue virus nonstructural protein NS1 early in dengue illness correlate with the development of dengue hemorrhagic fever. J Infect Dis. 2002;186(8):1165–8.
Article CAS PubMed Google Scholar
Bhatt P, Sabeena SP, Varma M, Arunkumar G. Current understanding of the pathogenesis of dengue virus infection. Curr Microbiol. 2021;78(1):17–32. https://doi.org/10.1007/s00284-020-02284-w .
Gutsche I, Coulibaly F, Voss JE, Salmon J, D’Alayer J, Ermonval M, et al. Secreted dengue virus nonstructural protein NS1 is an atypical barrel-shaped high-density lipoprotein. Proc Natl Acad Sci U S A. 2011;108(19):8003–8.
Modhiran N, Watterson D, Muller DA, Panetta AK, Sester DP, Liu L, et al. Dengue virus NS1 protein activates cells via Toll-like receptor 4 and disrupts endothelial cell monolayer integrity. Sci Transl Med. 2015;7:304.
Lee WHK, Liu W, Fan JS, Yang D. Dengue virus protease activity modulated by dynamics of protease cofactor. Biophys J. 2021;120(12):2444–53. https://doi.org/10.1016/j.bpj.2021.04.015 .
McLean JE, Wudzinska A, Datan E, Quaglino D, Zakeri Z. Flavivirus NS4A-induced autophagy protects cells against death and enhances virus replication. J Biol Chem. 2011;286(25):22147–59.
Henderson BR, Saeedi BJ, Campagnola G, Geiss BJ. Analysis of RNA binding by the dengue virus NS5 RNA capping enzyme. PLoS One. 2011;6(10):1–9.
Tay MYF, Fraser JE, Chan WKK, Moreland NJ, Rathore AP, Wang C, et al. Nuclear localization of dengue virus (DENV) 1–4 non-structural protein 5; protection against all 4 DENV serotypes by the inhibitor Ivermectin. Antiviral Res. 2013;99(3):301–6. https://doi.org/10.1016/j.antiviral.2013.06.002 .
Bressanelli S, Stiasny K, Allison SL, Stura EA, Duquerroy S, Lescar J, et al. Structure of a flavivirus envelope glycoprotein in its low-pH-induced membrane fusion conformation. EMBO J. 2004;23(4):728–38.
Bhatt S, Gething PW, Brady OJ, Messina JP, Farlow AW, Moyes CL, et al. The global distribution and burden of dengue. Nature. 2013;496(7446):504–7. https://doi.org/10.1038/nature12060 .
Dwivedi VD, Tripathi IP, Tripathi RC, Bharadwaj S, Mishra SK. Genomics, proteomics and evolution of dengue virus. Brief Funct Genomics. 2017;16(4):217–27.
CAS PubMed Google Scholar
Madhry D, Pandey KK, Kaur J, Rawat Y, Sapra L, Kumar RYS, et al. Role of non-coding RNAs in dengue virus-host interaction. Front Biosci Sch. 2021;13(1):44–55.
Article CAS Google Scholar
Guzman MG, Halstead SB, Artsob H, Buchy P, Farrar J, Nathan MB, et al. Europe PMC Funders Group Dengue: a continuing global threat Europe PMC Funders Author Manuscripts. Nat Rev Microbiol. 2010;8(12):7–16.
Muller DA, Young PR. The flavivirus NS1 protein: molecular and structural biology, immunology, role inpathogenesis and application asadiagnostic biomarker. Antiviral Res. 2013;98(2):192–208. https://doi.org/10.1016/j.antiviral.2013.03.008 .
Murphy BR, Whitehead SS. Immune response to dengue virus and prospects for a vaccine. Annu Rev Immunol. 2011;29:587–619.
Yacoub S, Mongkolsapaya J, Screaton G. The pathogenesis of dengue. Curr Opin Infect Dis. 2013;26(3):284–9.
Roy SK, Bhattacharjee S. Dengue virus: epidemiology, biology, and disease aetiology. Can J Microbiol. 2021;67(10):687–702.
Wong JM, Adams LE, Durbin AP, Muñoz-Jordán JL, Poehling KA, Sánchez-González LM, et al. Dengue: a growing problem with new interventions. Pediatrics. 2022;149(6):e2021055522.
Article PubMed Google Scholar
Diamond MS, Pierson TC. Molecular insight into dengue virus pathogenesis and its implications for disease control. Cell. 2015;162(3):488–92. https://doi.org/10.1016/j.cell.2015.07.005 .
Simmons CP, McPherson K, Van Vinh CN, Hoai Tam DT, Young P, Mackenzie J, et al. Recent advances in dengue pathogenesis and clinical management. Vaccine. 2015;33(50):7061–8.
Yong YK, Wong WF, Vignesh R, Chattopadhyay I, Velu V, Tan HY, et al. Dengue infection - recent advances in disease pathogenesis in the era of COVID-19. Front Immunol. 2022;13(July):1–17.
Palanichamy Kala M, St. John AL, Rathore APS. Dengue: update on clinically relevant therapeutic strategies and vaccines. Curr Treat Options Infect Dis. 2023;15(2):27–52. https://doi.org/10.1007/s40506-023-00263-w .
Article PubMed PubMed Central Google Scholar
Rothman AL. Immunity to dengue virus: a tale of original antigenic sin and tropical cytokine storms. Nat Rev Immunol. 2011;11(8):532–43.
Dinakaran D, Sreeraj VS, Venkatasubramanian G. Dengue and psychiatry: manifestations, mechanisms, and management options. Indian J Psychol Med. 2022;44(5):429–35.
Wang X, Xia H, Liu S, Cao L, You F. Epigenetic regulation in antiviral innate immunity. Eur J Immunol. 2021;51(7):1641–51.
Zhang Y, Guo J, Gao Y, Li S, Pan T, Xu G, et al. Dynamic transcriptome analyses reveal m6A regulated immune non-coding RNAs during dengue disease progression. Heliyon. 2023;9(1):e12690.
Gokhale NS, McIntyre ABR, McFadden MJ, Roder AE, Kennedy EM, Gandara JA, et al. N6-methyladenosine in flaviviridae viral RNA genomes regulates infection. Cell Host Microbe. 2016;20(5):654–65. https://doi.org/10.1016/j.chom.2016.09.015 .
Ruggieri A, Helm M, Chatel-Chaix L. An epigenetic ‘extreme makeover’: the methylation of flaviviral RNA (and beyond). RNA Biol. 2021;18(5):696–708. https://doi.org/10.1080/15476286.2020.1868150 .
Henry BA, Kanarek JP, Kotter A, Helm M, Lee N. 5-Methylcytosine modification of an Epstein-Barr virus noncoding RNA decreases its stability. RNA. 2020;26(8):1038–48.
Courtney DG, Chalem A, Bogerd HP, Law BA, Kennedy EM, Holley CL, et al. Extensive epitranscriptomic methylation of A and C residues on murine leukemia virus transcripts enhances viral gene expression. MBio. 2019;10(3):1–12.
Caraballo GI, Rosales R, Viettri M, Castillo JM, Cruz R, Ding S, et al. The dengue virus nonstructural protein 1 (NS1) interacts with the putative epigenetic regulator DIDO1 to promote flavivirus replication in mosquito cells. J Virol. 2022;96(12):1–17.
Colpitts TM, Barthel S, Wang P, Fikrig E. Dengue virus capsid protein binds core histones and inhibits nucleosome formation in human liver cells. PLoS One. 2011;6(9):e24365.
Banerjee A, Tripathi A, Duggal S, Banerjee A, Vrati S. Dengue virus infection impedes megakaryopoiesis in MEG-01 cells where the virus envelope protein interacts with the transcription factor TAL-1. Sci Rep. 2020;10(1):1–12. https://doi.org/10.1038/s41598-020-76350-5 .
Lahon A, Arya RP, Banerjea AC. Dengue virus dysregulates master transcription factors and PI3K/AKT/mTOR signaling pathway in megakaryocytes. Front Cell Infect Microbiol. 2021;11(August):1–11.
Butler M, Chotiwan N, Brewster CD, DiLisio JE, Ackart DF, Podell BK, et al. Cyclin-dependent kinases 8 and 19 regulate host cell metabolism during dengue virus serotype 2 infection. Viruses. 2020;12(6):1–21.
Zaborowska J, Isa NF, Murphy S. P-TEFb goes viral. BioEssays. 2016;38:S75-85.
De Maio FA, Risso G, Iglesias NG, Shah P, Pozzi B, Gebhard LG, et al. The dengue virus NS5 protein intrudes in the cellular spliceosome and modulates splicing. PLoS Pathog. 2016;12(8):1–29.
Pozzi B, Bragado L, Mammi P, Torti MF, Gaioli N, Gebhard LG, et al. Dengue virus targets RBM10 deregulating host cell splicing and innate immune response. Nucleic Acids Res. 2020;48(12):6824–38.
Dickson AM, Wilusz J. Strategies for viral RNA stability: live long and prosper. Trends Genet. 2011;27(7):286–93.
Balinsky CA, Schmeisser H, Wells AI, Ganesan S, Jin T, Singh K, Zoon KC. IRAV (FLJ11286), an interferonstimulated gene with antiviral activity against dengue virus, interacts with MOV10. J Virol. 2017;91(5):10–128.
Walsh D, Mohr I. Viral subversion of the host protein synthesis machinery. Nat Rev Microbiol. 2011;9(12):860–75.
Bidet K, Dadlani D, Garcia-Blanco MA. Correction: G3BP1, G3BP2 and CAPRIN1 are required for translation of interferon stimulated mRNAs and are targeted by a dengue virus non-coding RNA. PLoS Pathog. 2017;13(3):e1006295.
Polacek C, Friebe P, Harris E. Poly(A)-binding protein binds to the non-polyadenylated 3′ untranslated region of dengue virus and modulates translation efficiency. J Gen Virol. 2009;90(3):687–92.
Wang K, Zou C, Wang X, Huang C, Feng T, Pan W, et al. Interferon-stimulated TRIM69 interrupts dengue virus replication by ubiquitinating viral nonstructural protein 3. PLoS Pathog. 2018;14(8):1–24.
Pokidysheva E, Zhang Y, Battisti AJ, Bator-Kelly CM, Chipman PR, Xiao C, et al. Cryo-EM reconstruction of dengue virus in complex with the carbohydrate recognition domain of DC-SIGN. Cell. 2006;124(3):485–93.
Idris F, Muharram SH, Diah S. Glycosylation of dengue virus glycoproteins and their interactions with carbohydrate receptors: possible targets for antiviral therapy. Arch Virol. 2016;161(7):1751–60.
Yap SSL, Nguyen-Khuong T, Rudd PM, Alonso S. Dengue virus glycosylation: what do we know? Front Microbiol. 2017;8(JUL):1–16.
CAS Google Scholar
Thiemmeca S, Tamdet C, Punyadee N, Prommool T, Songjaeng A, Noisakran S, et al. Secreted NS1 protects dengue virus from mannose-binding lectin-mediated neutralization. J Immunol. 2016;197(10):4053–65.
Ashour J, Laurent-Rolle M, Shi P-Y, García-Sastre A. NS5 of dengue virus mediates STAT2 binding and degradation. J Virol. 2009;83(11):5408–18.
Srivastava S, Chaudhary N, Ojha A, Guchhait P, Patel AK. Signal transducer and activator of transcription 3 (STAT3) acts as a proviral factor for dengue virus propagation. Virus Res. 2021;300(March):198436. https://doi.org/10.1016/j.virusres.2021.198436 .
Emara MM, Brinton MA. Interaction of TIA-1/TIAR with West Nile and dengue virus products in infected cells interferes with stress granule formation and processing body assembly. Proc Natl Acad Sci U S A. 2007;104(21):9041–6.
Ward AM, Bidet K, Yinglin A, Ler SG, Hogue K, Blackstock W, et al. Quantitative mass spectrometry of DENV-2 RNA-interacting proteins reveals that the DEAD-box RNA helicase DDX6 binds the DB1 and DB2 3’ UTR structures. RNA Biol. 2011;8(6):1173–86.
Arakawa M, Tabata K, Ishida K, Kobayashi M, Arai A, Ishikawa T, et al. Flavivirus recruits the valosin-containing protein-NPL4 complex to induce stress granule disassembly for efficient viral genome replication. J Biol Chem. 2022;298(3):101597. https://doi.org/10.1016/j.jbc.2022.101597 .
Mishra R, Lahon A, Banerjea AC. Dengue virus degrades USP33–ATF3 axis via extracellular vesicles to activate human microglial cells. J Immunol. 2020;205(7):1787–98.
De Aguiar GPCG, Da Silva Leite CMG, Dias B, Vasconcelos SMM, De Moraes RA, De Moraes MEA, et al. Evidence for host epigenetic signatures arising from arbovirus infections: a systematic review. Front Immunol. 2019;10(MAY):1–14.
Bartholomeusz A, Locarnini S. Associated with antiviral therapy. Antivir Ther. 2006;55(November 2005):52–5.
Shah PS, Link N, Jang GM, Sharp PP, Zhu T, Swaney DL, et al. Comparative flavivirus-host protein interaction mapping reveals mechanisms of dengue and Zika virus pathogenesis. Cell. 2018;175(7):1931-1945.e18.
Balinsky CA, Schmeisser H, Ganesan S, Singh K, Pierson TC, Zoon KC. Nucleolin interacts with the dengue virus capsid protein and plays a role in formation of infectious virus particles. J Virol. 2013;87(24):13094–106.
Li L, Li J, Gao M, Fan H, Wang Y, Xu X, et al. Interleukin-8 as a biomarker for disease prognosis of coronavirus disease-2019 patients. Front Immunol. 2021;11(January):1–10.
Murayama T, Kuno K, Jisaki F, Obuchi M, Sakamuro D, Furukawa T, Mukaida N, Matsushima K. Enhancement human cytomegalovirus replication in a human lung fibroblast cell line by interleukin-8. J Virol. 1994;68(11):7582–5.
Raghupathy R, Chaturvedi UC, Al-Sayer H, Elbishbishi EA, Agarwal R, Nagar R, et al. Elevated levels of IL-8 in dengue hemorrhagic fever. J Med Virol. 1998;56(3):280–5.
Li LL, Hu ST, Wang SH, Lee HH, Wang YT, Ping YH. Positive transcription elongation factor b (P-TEFb) contributes to dengue virus-stimulated induction of interleukin-8 (IL-8). Cell Microbiol. 2010;12(11):1589–603.
Wang X, Zhu J, Zhang D, Liu G. Ribosomal control in RNA virus-infected cells. Front Microbiol. 2022;13(November):1–15.
Weng SC, Tsao PN, Shiao SH. Blood glucose promotes dengue virus infection in the mosquito Aedes aegypti. Parasit Vectors. 2021;14(1):1–9. https://doi.org/10.1186/s13071-021-04877-1 .
Fontaine KA, Sanchez EL, Camarda R, Lagunoff M. Dengue virus induces and requires glycolysis for optimal replication. J Virol. 2015;89(4):2358–66.
Carlin AF, Plummer EM, Vizcarra EA, Sheets N, Joo Y, Tang W, et al. An IRF-3-, IRF-5-, and IRF-7-independent pathway of dengue viral resistance utilizes IRF-1 to stimulate type I and II interferon responses. Cell Rep. 2017;21(6):1600–12. https://doi.org/10.1016/j.celrep.2017.10.054 .
Ferrari M, Zevini A, Palermo E, Muscolini M, Alexandridi M, Etna MP, et al. Dengue virus targets Nrf2 for NS2B3-mediated degradation leading to enhanced oxidative stress and viral replication. J Virol. 2020;94(24):e01551-20.
Kaur J, Rawat Y, Sood V, Periwal N, Rathore DK, Kumar S, et al. Replication of dengue virus in K562-megakaryocytes induces suppression in the accumulation of reactive oxygen species. Front Microbiol. 2022;12(January):1–16.
Simon AY, Sutherland MR, Pryzdial ELG. Dengue virus binding and replication by platelets. Blood. 2015;126(3):378–85.
Han J, Xiong J, Wang D, Fu XD. Pre-mRNA splicing: where and when in the nucleus. Trends Cell Biol. 2011;21(6):336–43. https://doi.org/10.1016/j.tcb.2011.03.003 .
Chauhan K, Kalam H, Dutt R, Kumar D. RNA splicing: a new paradigm in host-pathogen interactions. J Mol Biol. 2019;431(8):1565–75. https://doi.org/10.1016/j.jmb.2019.03.001 .
Ashraf U, Benoit-Pilven C, Lacroix V, Navratil V, Naffakh N. Advances in analyzing virus-induced alterations of host cell splicing. Trends Microbiol. 2019;27(3):268–81. https://doi.org/10.1016/j.tim.2018.11.004 .
Boudreault S, Roy P, Lemay G, Bisaillon M. Viral modulation of cellular RNA alternative splicing: a new key player in virus–host interactions? Wiley Interdiscip Rev RNA. 2019;10(5):1–20.
Lightfoot HL, Hall J. Endogenous polyamine function - the RNA perspective. Nucleic Acids Res. 2014;42(18):11275–90.
Miller-Fleming L, Olin-Sandoval V, Campbell K, Ralser M. Remaining mysteries of molecular biology: the role of polyamines in the cell. J Mol Biol. 2015;427(21):3389–406. https://doi.org/10.1016/j.jmb.2015.06.020 .
Mounce BC, Cesaro T, Moratorio G, Hooikaas PJ, Yakovleva A, Werneke SW, et al. Inhibition of polyamine biosynthesis is a broad-spectrum strategy against RNA viruses. J Virol. 2016;90(21):9683–92.
Li MMH, MacDonald MR. Polyamines: small molecules with a big role in promoting virus infection. Cell Host Microbe. 2016;20(2):123–4. https://doi.org/10.1016/j.chom.2016.07.012 .
Mounce BC, Poirier EZ, Passoni G, Simon-Loriere E, Cesaro T, Prot M, et al. Interferon-induced spermidine-spermine acetyltransferase and polyamine depletion restrict Zika and Chikungunya viruses. Cell Host Microbe. 2016;20(2):167–77. https://doi.org/10.1016/j.chom.2016.06.011 .
Michalski D, Gustavo Ontiveros J, Russo J, Charley PA, Anderson JR, Heck AM, et al. Zika virus noncoding sfRNAs sequester multiple host-derived RNA-binding proteins and modulate mRNA decay and splicing during infection. J Biol Chem. 2019;294(44):16282–96.
Petit MJ, Kenaston MW, Pham OH, Nagainis AA, Fishburn AT, Shah PS. Nuclear dengue virus NS5 antagonizes expression of PAF1-dependent immune response genes. PLoS Pathog. 2021;17(11):1–23. https://doi.org/10.1371/journal.ppat.1010100 .
Clyde K, Kyle JL, Harris E. Recent advances in deciphering viral and host determinants of dengue virus replication and pathogenesis. J Virol. 2006;80(23):11418–31.
Shukla R, Ramasamy V, Shanmugam RK, Ahuja R, Khanna N. Antibody-dependent enhancement: a challenge for developing a safe dengue vaccine. Front Cell Infect Microbiol. 2020;10(October):1–12.
Narayan R, Tripathi S. Intrinsic ADE: the dark side of antibody dependent enhancement during dengue infection. Front Cell Infect Microbiol. 2020;10(October):1–6.
Viktorovskaya OV, Greco TM, Cristea IM, Thompson SR. Identification of RNA binding proteins associated with dengue virus RNA in infected cells reveals temporally distinct host factor requirements. PLoS Negl Trop Dis. 2016;10(8):1–22.
Abernathy E, Glaunsinger B. Emerging roles for RNA degradation in viral replication and antiviral defense. Virology. 2015;479–480:600–8. https://doi.org/10.1016/j.virol.2015.02.007 .
Ahmed MR, Du Z. Molecular interaction of nonsense-mediated mRNA decay with viruses. Viruses. 2023;15(4):816.
Kakumani PK, Rajgokul KS, Ponia SS, Kaur I, Mahanty S, Medigeshi GR, et al. Dengue NS3, an RNAi suppressor, modulates the human miRNA pathways through its interacting partner. Biochem J. 2015;471(1):89–99.
Richter JD, Sonenberg N. Regulation of cap-dependent translation by eIF4E inhibitory proteins. Nature. 2005;433(7025):477–80.
Villas-Bôas CSA, Conceição TM, Ramírez J, Santoro ABM, Da Poian AT, Montero-Lomelí M. Dengue virus-induced regulation of the host cell translational machinery. Brazilian J Med Biol Res. 2009;42(11):1020–6.
Bidet K, Garcia-Blanco MA. Flaviviral RNAs: weapons and targets in the war between virus and host. Biochem J. 2014;462(2):215–30.
Morais ATS, Terzian ACB, Duarte DVB, Bronzoni RVB, Madrid MCFS, Gavioli AF, et al. The eukaryotic translation initiation factor 3 subunit L protein interacts with Flavivirus NS5 and may modulate yellow fever virus replication. Virol J. 2013;10(1):1.
Davis WG, Blackwell JL, Shi P-Y, Brinton MA. Interaction between the cellular protein eEF1A and the 3′-terminal stem-loop of West Nile virus genomic RNA facilitates viral minus-strand RNA synthesis. J Virol. 2007;81(18):10172–87.
Holden KL, Harris E. Enhancement of dengue virus translation: role of the 3′ untranslated region and the terminal 3′ stem-loop domain. Virology. 2004;329(1):119–33.
Edgil D, Polacek C, Harris E. Dengue virus utilizes a novel strategy for translation initiation when cap-dependent translation is inhibited. J Virol. 2006;80(6):2976–86.
Kieft JS, Zhou K, Jubin R, Doudna JA. Mechanism of ribosome recruitment by hepatitis C IRES RNA. RNA. 2001;7(2):194–206.
Verma B, Bhattacharyya S, Das S. Polypyrimidine tract-binding protein interacts with coxsackievirus B3 RNA and influences its translation. J Gen Virol. 2010;91(5):1245–55.
Zeng L, Falgout B, Markoff L. Identification of specific nucleotide sequences within the conserved 3′-SL in the dengue type 2 virus genome required for replication. J Virol. 1998;72(9):7510–22.
Bhattacharyya S, Verma B, Pandey G, Das S. The structure and function of a cis-acting element located upstream of the IRES that influences Coxsackievirus B3 RNA translation. Virology. 2008;377(2):345–54.
Kumar R, Mehta D, Mishra N, Nayak D, Sunil S. Role of host-mediated post-translational modifications (PTMS) in RNA virus pathogenesis. Int J Mol Sci. 2021;22(1):1–26.
Aguirre S, Luthra P, Sanchez-Aparicio MT, Maestre AM, Patel J, Lamothe F, et al. Dengue virus NS2B protein targets cGAS for degradation and prevents mitochondrial DNA sensing during infection. Nat Microbiol. 2017;2(March):1–11.
Aguirre S, Maestre AM, Pagni S, Patel JR, Savage T, Gutman D, et al. DENV inhibits type I IFN production in infected cells by cleaving human STING. PLoS Pathog. 2012;8(10):e1002934.
Mazzon M, Jones M, Davidson A, Chain B, Jacobs M. Dengue virus ns5 inhibits interferon-a signaling by blocking signal transducer and activator of transcription 2 phosphorylation. J Infect Dis. 2009;200(8):1261–70.
Zhang J, Lan Y, Li MY, Lamers MM, Fusade-Boyer M, Klemm E, et al. Flaviviruses exploit the lipid droplet protein AUP1 to trigger lipophagy and drive virus production. Cell Host Microbe. 2018;23(6):819-831.e5. https://doi.org/10.1016/j.chom.2018.05.005 .
Ye J, Zhang H, He W, Zhu B, Zhou D, Chen Z, et al. Quantitative phosphoproteomic analysis identifies the critical role of JNK1 in neuroinflammation induced by Japanese encephalitis virus. Sci Signal. 2016;9(448):1–16.
Zhang H, Sun J, Ye J, Ashraf U, Chen Z, Zhu B, et al. Quantitative label-free phosphoproteomics reveals differentially regulated protein phosphorylation involved in West Nile virus-induced host inflammatory response. J Proteome Res. 2015;14(12):5157–68.
Scaturro P, Stukalov A, Haas DA, Cortese M, Draganova K, Płaszczyca A, et al. An orthogonal proteomic survey uncovers novel Zika virus host factors. Nature. 2018;561(7722):253–7.
Guan Y, Wang Y, Fu X, Bai G, Li X, Mao J, et al. Multiple functions of stress granules in viral infection at a glance. Front Microbiol. 2023;14(March):1–11.
Anderson P, Kedersha N. RNA granules: post-transcriptional and epigenetic modulators of gene expression. Nat Rev Mol Cell Biol. 2009;10(6):430–6. https://doi.org/10.1038/nrm2694 .
Xia J, Chen X, Xu F, Wang Y, Shi Y, Li Y, et al. Dengue virus infection induces formation of G3BP1 granules in human lung epithelial cells. Arch Virol. 2015;160(12):2991–9.
Hou S, Kumar A, Xu Z, Airo AM, Stryapunina I. crossm Zika virus hijacks stress granule proteins. J Virol. 2017;91(16):1–21.
Katoh H, Okamoto T, Fukuhara T, Kambara H, Morita E, Mori Y, et al. Japanese encephalitis virus core protein inhibits stress granule formation through an interaction with Caprin-1 and facilitates viral propagation. J Virol. 2013;87(1):489–502.
Madhry D, Malvankar S, Phadnis S, Srivastava RK, Bhattacharyya S, Verma B. Synergistic correlation between host angiogenin and dengue virus replication. RNA Biol. 2023;20(1):805–16. https://doi.org/10.1080/15476286.2023.2264003 .
Pandey KK, Madhry D, Ravi Kumar YS, Malvankar S, Sapra L, Srivastava RK, et al. Regulatory roles of tRNA-derived RNA fragments in human pathophysiology. Mol Ther - Nucleic Acids. 2021;26(December):161–73.
Diwaker D, Mishra KP, Ganju L. Effect of modulation of unfolded protein response pathway on dengue virus infection. Acta Biochim Biophys Sin (Shanghai). 2015;47(12):960–8.
Peña J, Harris E. Dengue virus modulates the unfolded protein response in a time-dependent manner. J Biol Chem. 2011;286(16):14226–36.
Silva JP, Fernandez-Sesma A. Challenges on the development of a dengue vaccine: a comprehensive review of the state of the art. J Gen Virol. 2023;104(3):1–12.
Obi JO, Gutiérrez-Barbosa H, Chua JV, Deredge DJ. Current trends and limitations in dengue antiviral research. Trop Med Infect Dis. 2021;6(4):1–19.
Goethals O, Kaptein SJF, Kesteleyn B, Bonfanti JF, Van Wesenbeeck L, Bardiot D, et al. Blocking NS3–NS4B interaction inhibits dengue virus in non-human primates. Nature. 2023;615(7953):678–86.
Kaptein SJF, Goethals O, Kiemel D, Marchand A, Kesteleyn B, Bonfanti JF, et al. Publisher Correction: A pan-serotype dengue virus inhibitor targeting the NS3–NS4B interaction (Nature, (2021), 598, 7881, (504-509), 10.1038/s41586-021-03990-6). Nature. 2021;599(7883):E2.
Xie X, Wang Q-Y, Xu HY, Qing M, Kramer L, Yuan Z, et al. Inhibition of dengue virus by targeting viral NS4B protein. J Virol. 2011;85(21):11183–95.
van Cleef KWR, Overheul GJ, Thomassen MC, Kaptein SJF, Davidson AD, Jacobs M, et al. Identification of a new dengue virus inhibitor that targets the viral NS4B protein and restricts genomic RNA replication. Antiviral Res. 2013;99(2):165–71. https://doi.org/10.1016/j.antiviral.2013.05.011 .
Van Cleef KWR, Overheul GJ, Thomassen MC, Marjakangas JM, Van Rij RP. Escape mutations in NS4B render dengue virus insensitive to the antiviral activity of the paracetamol metabolite AM404. Antimicrob Agents Chemother. 2016;60(4):2554–7.
Wang Q-Y, Dong H, Zou B, Karuna R, Wan KF, Zou J, et al. Discovery of dengue virus NS4B inhibitors. J Virol. 2015;89(16):8233–44.
Moquin SA, Simon O, Karuna R, Lakshminarayana SB, Yokokawa F, Wang F, et al. NITD-688, a pan-serotype inhibitor ofthe dengue virus NS4B protein, shows favorable pharmacokinetics and efficacy in preclinical animal models. Sci Transl Med. 2021;13(579):1–14.
Songprakhon P, Thaingtamtanha T, Limjindaporn T, Puttikhunt C, Srisawat C, Luangaram P, et al. Peptides targeting dengue viral nonstructural protein 1 inhibit dengue virus production. Sci Rep. 2020;10(1):1–16. https://doi.org/10.1038/s41598-020-69515-9 .
Schmidt AG, Lee K, Yang PL, Harrison SC. Small-molecule inhibitors of dengue-virus entry. PLoS Pathog. 2012;8(4):e1002627.
Hrobowski YM, Garry RF, Michael SF. Peptide inhibitors of dengue virus and West Nile virus infectivity. Virol J. 2005;2:1–10.
Wang QY, Patel SJ, Vangrevelinghe E, Hao YX, Rao R, Jaber D, et al. A small-molecule dengue virus entry inhibitor. Antimicrob Agents Chemother. 2009;53(5):1823–31.
Abdul Ahmad SA, Palanisamy UD, Tejo BA, Chew MF, Tham HW, Syed HS. Geraniin extracted from the rind of Nephelium lappaceum binds to dengue virus type-2 envelope protein and inhibits early stage of virus replication. Virol J. 2017;14(1):1–13.
Rothan HA, Abdulrahman AY, Sasikumer PG, Othman S, Abd Rahman N, Yusof R. Protegrin-1 inhibits dengue NS2B-NS3 serine protease and viral replication in MK2 cells. J Biomed Biotechnol. 2012;2012:1–6.
Rothan HA, Han HC, Ramasamy TS, Othman S, Rahman NA, Yusof R. Inhibition of dengue NS2B-NS3 protease and viral replication in Vero cells by recombinant retrocyclin-1. BMC Infect Dis. 2012;12(1):1.
Tomlinson SM, Malmstrom RD, Russo A, Mueller N, Pang YP, Watowich SJ. Structure-based discovery of dengue virus protease inhibitors. Antiviral Res. 2009;82(3):110–4.
Lee MF, Wu YS, Poh CL. Molecular mechanisms of antiviral agents against dengue virus. Viruses. 2023;15(3):705.
Smith JL, Sheridan K, Parkins CJ, Frueh L, Jemison AL, Strode K, et al. Characterization and structure-activity relationship analysis of a class of antiviral compounds that directly bind dengue virus capsid protein and are incorporated into virions. Antiviral Res. 2018;155:12–9.
Xia H, Xie X, Zou J, Noble CG, Russell WK, Holthauzen LMF, et al. A cocrystal structure of dengue capsid protein in complex of inhibitor. Proc Natl Acad Sci U S A. 2020;117(30):17992–8001.
Suputtamongkol Y, Avirutnan P, Mairiang D, Angkasekwinai N, Niwattayakul K, Yamasmith E, et al. Ivermectin accelerates circulating nonstructural protein 1 (NS1) clearance in adult dengue patients: a combined phase 2/3 randomized double-blinded placebo controlled trial. Clin Infect Dis. 2021;72(10):E586–93.
Byrd CM, Grosenbach DW, Berhanu A, Dai D, Jones KF, Cardwell KB, et al. Novel benzoxazole inhibitor of dengue virus replication that targets the NS3 helicase. Antimicrob Agents Chemother. 2013;57(4):1902–12.
Basavannacharya C, Vasudevan SG. Suramin inhibits helicase activity of NS3 protein of dengue virus in a fluorescence-based high throughput assay format. Biochem Biophys Res Commun. 2014;453(3):539–44. https://doi.org/10.1016/j.bbrc.2014.09.113 .
Panya A, Sawasdee N, Junking M, Srisawat C, Choowongkomon K, Yenchitsomanus PT. A peptide inhibitor derived from the conserved ectodomain region of DENV membrane (M) protein with activity against dengue virus infection. Chem Biol Drug Des. 2015;86(5):1093–104.
Callahanid M, Trestonid AM, Lin G, Smith M, Kaufman B, Khaliq M, et al. Randomized single oral dose phase 1 study of safety, tolerability, and pharmacokinetics of Iminosugar UV-4 Hydrochloride (UV-4B) in healthy subjects. PLoS Negl Trop Dis. 2022;16(8):1–21. https://doi.org/10.1371/journal.pntd.0010636 .
Good SS, Shannon A, Lin K, Moussa A, Julander JG, La Colla P, et al. Evaluation of AT-752, a double prodrug of a guanosine nucleotide analog with in vitro and in vivo activity against dengue and other flaviviruses. Antimicrob Agents Chemother. 2021;65(11):e0098821.
Glasner DR, Ratnasiri K, Puerta-Guardo H, Espinosa DA, Beatty PR, Harris E. Dengue virus NS1 cytokine-independent vascular leak is dependent on endothelial glycocalyx components. PLoS Pathog. 2017;13(11):1–22.
St John AL, Rathore APS, Raghavan B, Ng ML, Abraham SN. Contributions of mast cells and vasoactive products, leukotrienes and chymase, to dengue virus-induced vascular leakage. Elife. 2013;2013(2):1–18.
Byrd CM, Dai D, Grosenbach DW, Berhanu A, Jones KF, Cardwell KB, et al. A novel inhibitor of dengue virus replication that targets the capsid protein. Antimicrob Agents Chemother. 2013;57(1):15–25.
Download references
Acknowledgements
The authors acknowledge All India Institute of Medical Sciences, New Delhi, and Department of Biotechnology, AIIMS, New Delhi for the support.
This work was supported to BV from SERB (EEQ/2022/000362), DBT (BT/PR39859/MED/29/1519/2020). The BV lab is also supported by research grants provided by AIIMS (AC-31, A-948).
Author information
Authors and affiliations.
Department of Biotechnology, All India Institute of Medical Sciences, Ansari Nagar, New Delhi, 110029, India
Saumya Sinha, Kinjal Singh, Riya Roy, Sushant Phadnis, Varsha Meena & Bhupendra Verma
Department of Biotechnology, M. S. Ramaiah Institute of Technology, MSR Nagar, Bengaluru, India
Y. S. Ravi Kumar
Translational Health Science and Technology Institute, NCR Biotech Science Cluster, Faridabad, India
Sankar Bhattacharyya
You can also search for this author in PubMed Google Scholar
Contributions
SS, KS, RKYS, and B.V. contributed to the conception, design, analysis, and drafting of the manuscript. RR, SP, VM and SB contributed to the drafting of the manuscript. All authors approved the final version of the manuscript for submission.
Corresponding author
Correspondence to Bhupendra Verma .
Ethics declarations
Ethics approval and consent to participate, consent for publication, competing interests.
The authors declare that they have no competing interests.
Additional information
Publisher’s note.
Springer Nature remains neutral with regard to jurisdictional claims in published maps and institutional affiliations.
Rights and permissions
Open Access This article is licensed under a Creative Commons Attribution 4.0 International License, which permits use, sharing, adaptation, distribution and reproduction in any medium or format, as long as you give appropriate credit to the original author(s) and the source, provide a link to the Creative Commons licence, and indicate if changes were made. The images or other third party material in this article are included in the article's Creative Commons licence, unless indicated otherwise in a credit line to the material. If material is not included in the article's Creative Commons licence and your intended use is not permitted by statutory regulation or exceeds the permitted use, you will need to obtain permission directly from the copyright holder. To view a copy of this licence, visit http://creativecommons.org/licenses/by/4.0/ . The Creative Commons Public Domain Dedication waiver ( http://creativecommons.org/publicdomain/zero/1.0/ ) applies to the data made available in this article, unless otherwise stated in a credit line to the data.
Reprints and permissions
About this article
Cite this article.
Sinha, S., Singh, K., Ravi Kumar, Y.S. et al. Dengue virus pathogenesis and host molecular machineries. J Biomed Sci 31 , 43 (2024). https://doi.org/10.1186/s12929-024-01030-9
Download citation
Received : 24 January 2024
Accepted : 14 April 2024
Published : 22 April 2024
DOI : https://doi.org/10.1186/s12929-024-01030-9
Share this article
Anyone you share the following link with will be able to read this content:
Sorry, a shareable link is not currently available for this article.
Provided by the Springer Nature SharedIt content-sharing initiative
- Dengue virus
- Epigenomic regulation
- Transcription regulation
- Post-transcriptional modifications
- Translation regulation
- Stress granule formation
Journal of Biomedical Science
ISSN: 1423-0127
- Submission enquiries: Access here and click Contact Us
- General enquiries: [email protected]
Dengue cases surge by nearly 50% in Americas amid 'emergency situation', UN agency says
- Medium Text
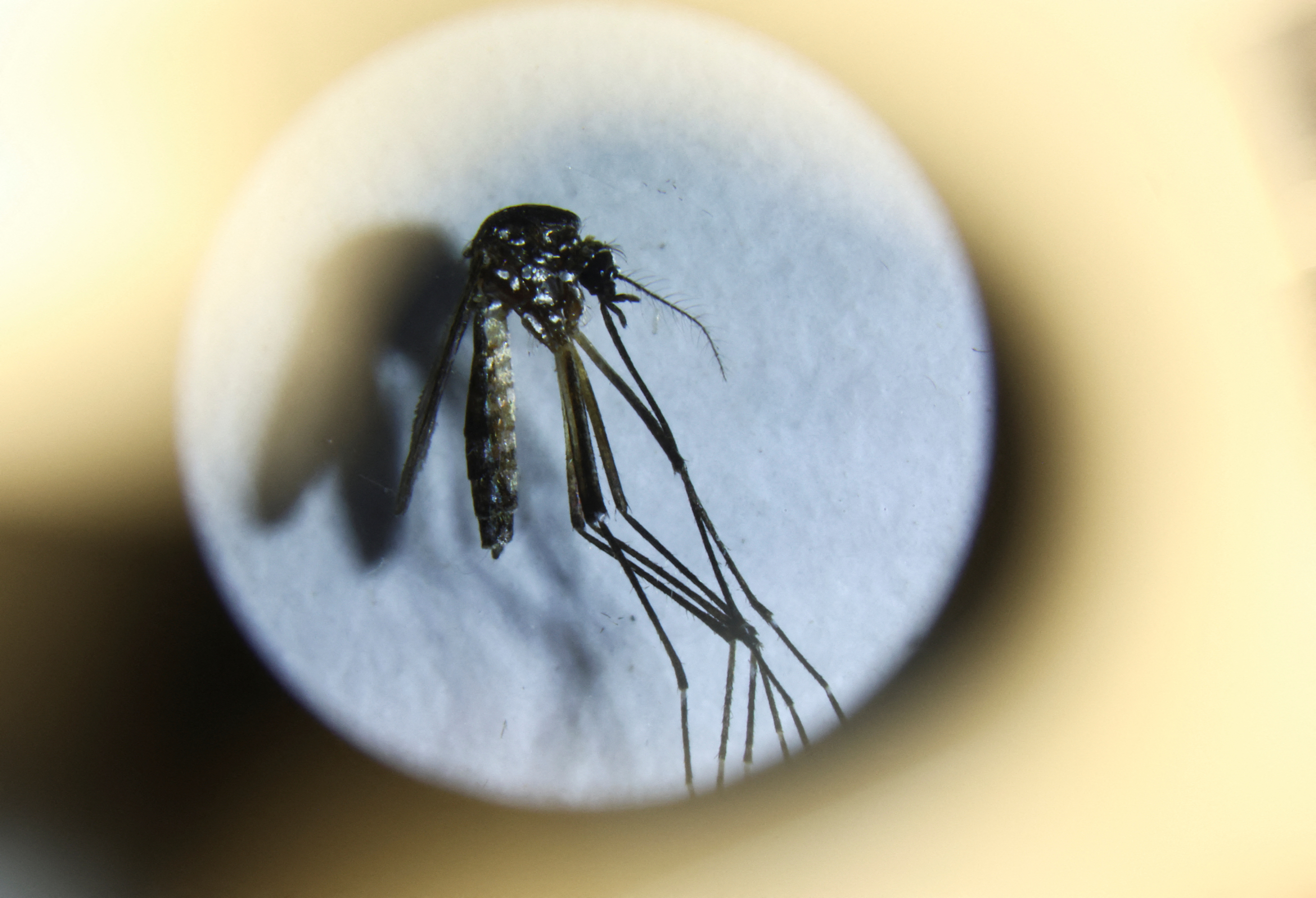
Sign up here.
Reporting by Brendan O'Boyle; Editing by Steven Grattan and Aurora Ellis
Our Standards: The Thomson Reuters Trust Principles. New Tab , opens new tab
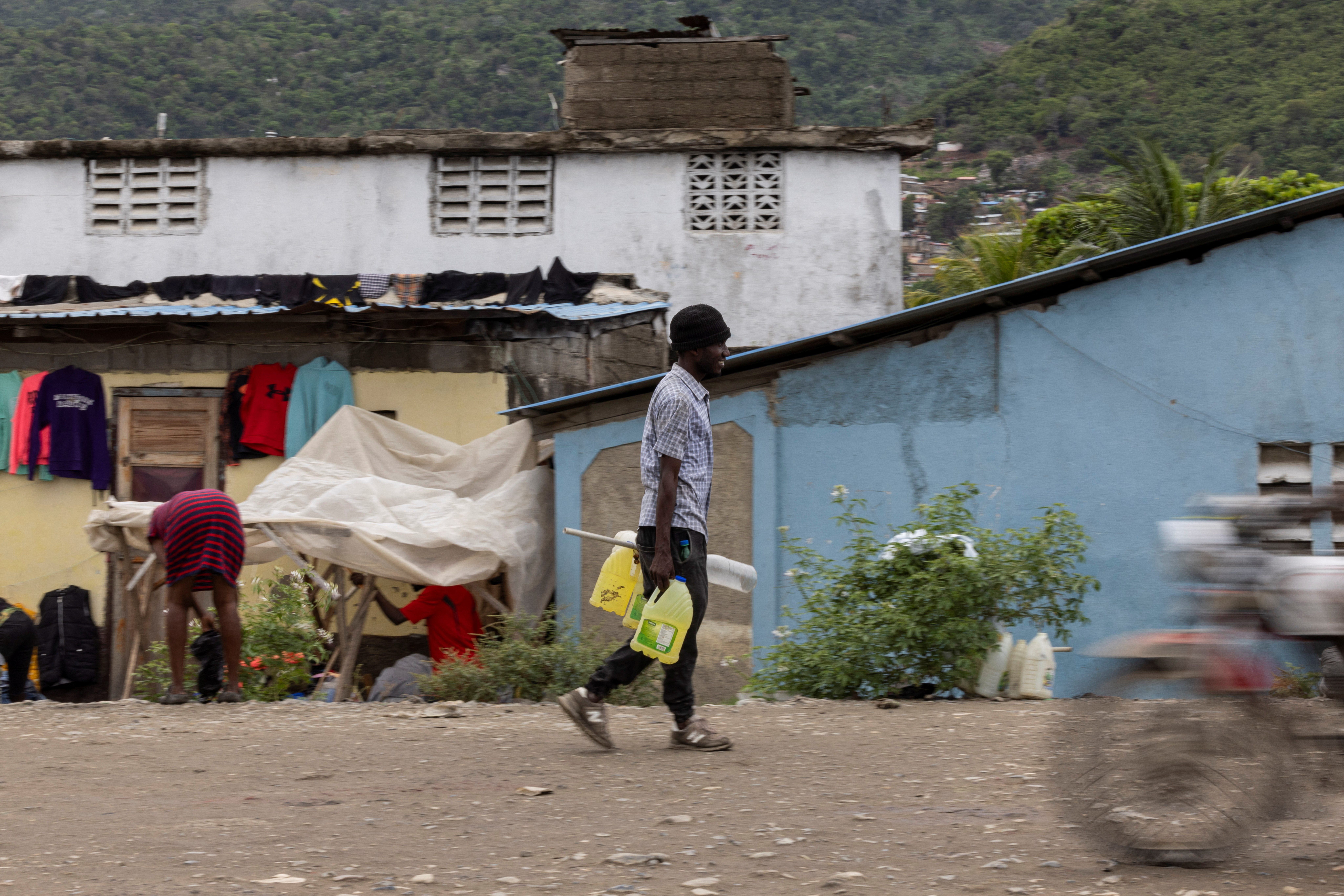
The United States on Monday blasted a Cuban court's decision to sentence a group of protesters to as many as 15 years in jail, calling the recent judgment "unconscionable" and "outrageous."

World Chevron
China's coast guard said on Monday it "expelled" a Philippine coast guard ship and another vessel from waters adjacent to the Scarborough Shoal, Chinese state media reported.
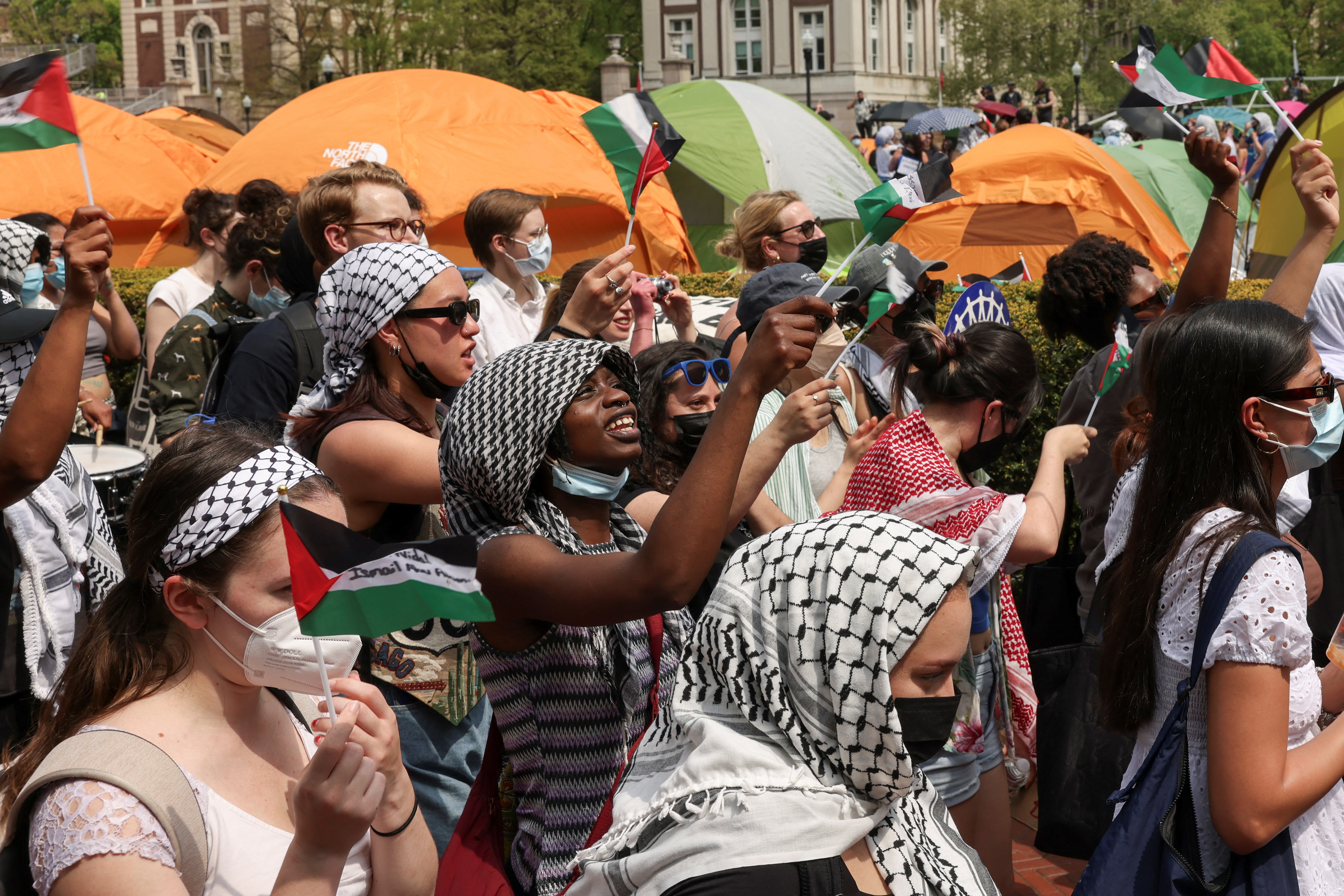
Four law enforcement officers were shot to death and four others were wounded on Monday in a gun battle that erupted while they were serving a fugitive arrest warrant at a house in Charlotte, North Carolina, authorities said.
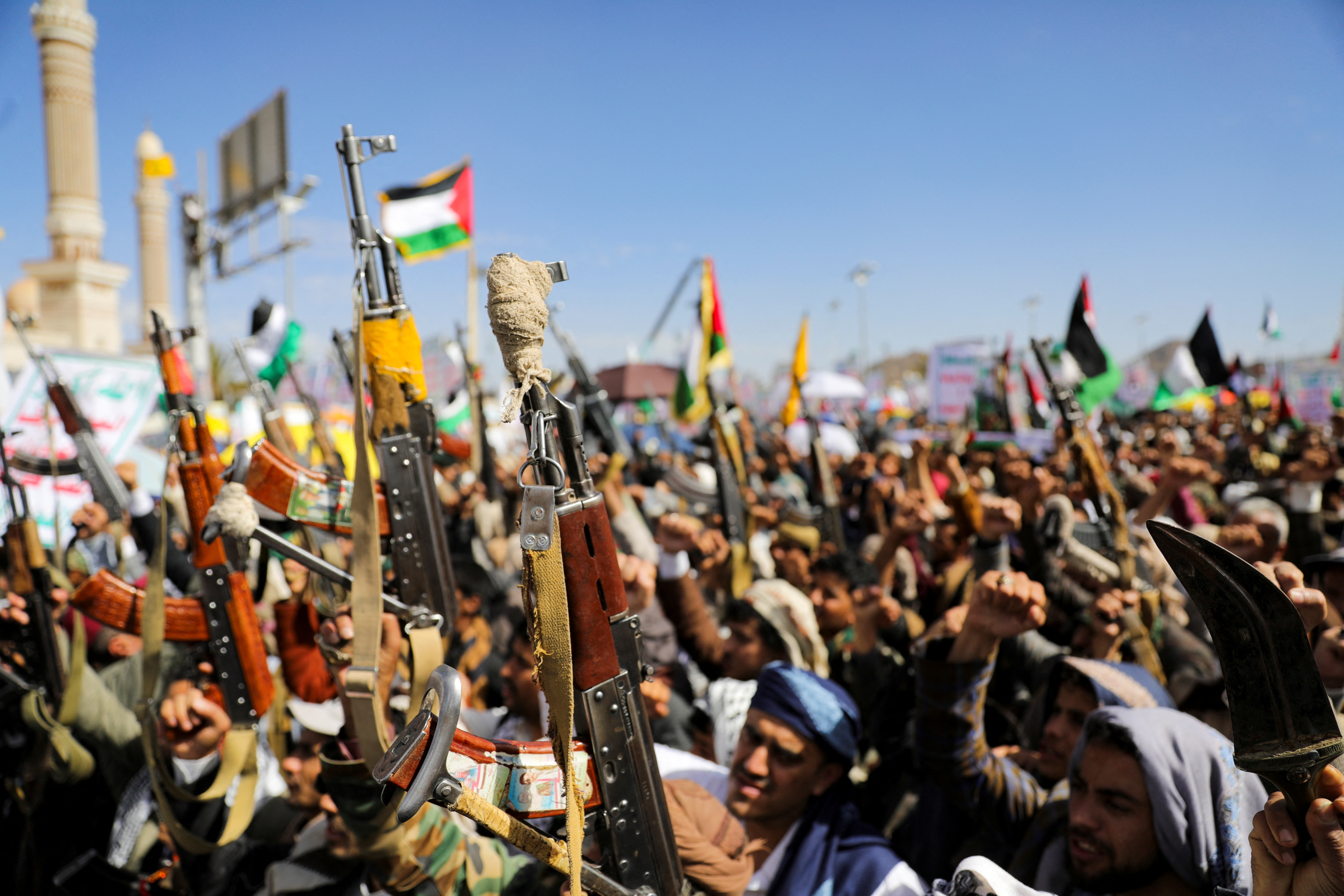
France warns of surge in imported dengue cases ahead of Olympics
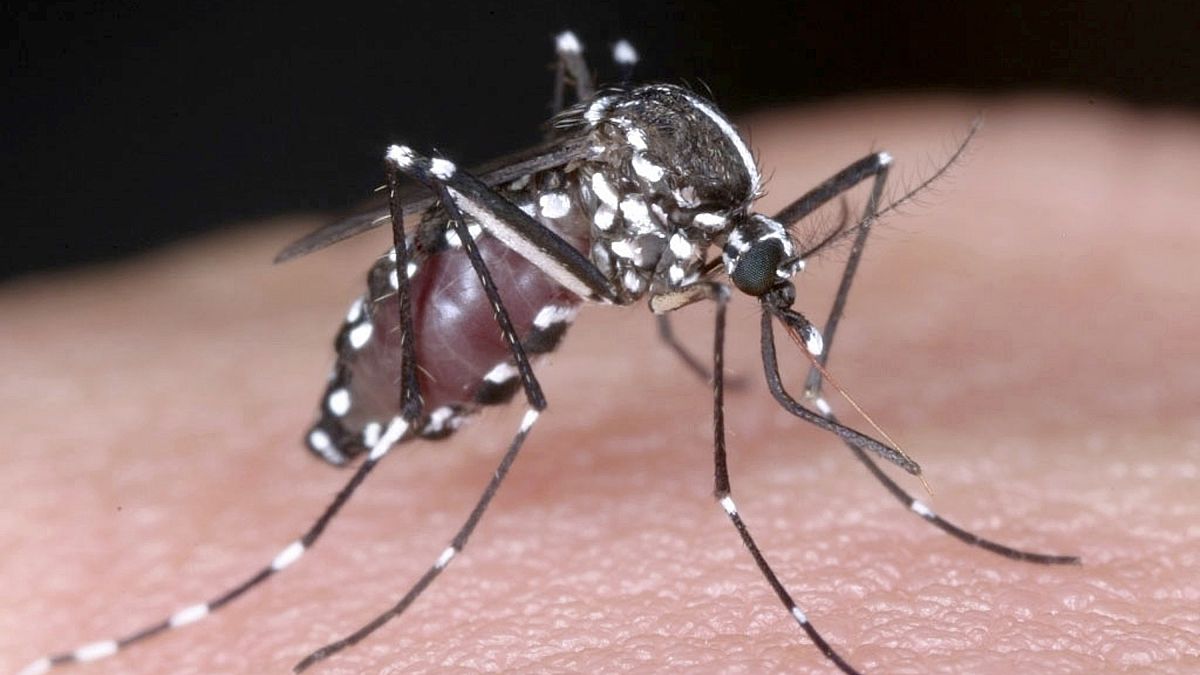
Mosquitoes spread the virus by biting an infected person and spreading it to another person through their bites.
French health authorities warn of the risks of imported cases of dengue fever ahead of the Olympics due to the ongoing surge of the virus in the Americas.
There are more than 5.2 million cases of dengue in the Americas, according to the World Health Organization (WHO)'s regional office, with many countries facing large epidemics.
This is up more than 400 per cent compared to the average over the past five years.
Dengue is a virus that is transmitted to humans through the bite of an infected mosquito. While many infections are asymptomatic, in some cases the virus can be severe or fatal.
The "unprecedented situation" in the Americas has resulted in an increase in imported cases from the region, French health authorities warned this week.
There have been more than 1,649 imported cases of dengue in mainland France, mostly coming from French overseas territories of Guadeloupe and Martinique.
This compared to just 131 cases over the same period last year, the country's health ministry said.
"The phenomenon that we observe [in France], with the rise in imported cases is a phenomenon that many countries have also observed," an official from the health ministry told a press briefing on Tuesday.
Warning ahead of the Olympics
French authorities called on health professionals and the public to be vigilant, especially as the tiger mosquito that transmits the dengue virus has expanded its presence in Europe.
The surveillance period in France begins from May to November for cases in the territory.
The concern is that a person infected with dengue can transmit it to a mosquito, which can in turn transmit the virus to someone else. It cannot be transmitted person-to-person, however.
A French health ministry official added the authorities were "fully mobilised" and preparing for any possible infectious disease threats during the Olympics.
When travelling to regions with high levels of dengue, people should wear loose clothes that cover the skin and try to prevent mosquito bites by using insect repellent and mosquito nets, authorities say.
When returning from those areas, Public Health France recommends consulting a doctor if you get a fever within 15 days and continuing to protect yourself from mosquitoes.
You might also like
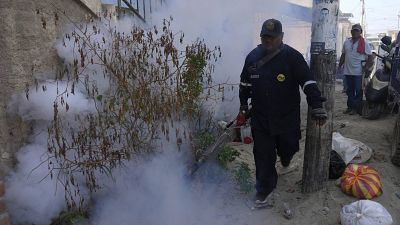
Peru declares health emergency in most provinces as dengue cases soar
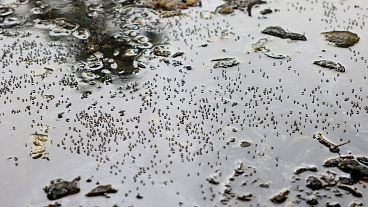
Dengue fever is likely to 'take off' in Europe as planet heats
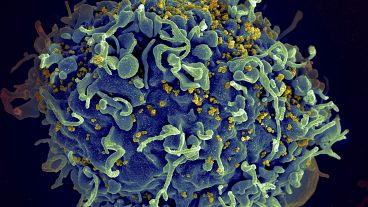
3 HIV cases linked to 'vampire facials' at US spa, CDC says
TheJakartaPost
Please Update your browser
Your browser is out of date, and may not be compatible with our website. A list of the most popular web browsers can be found below. Just click on the icons to get to the download page.
- Destinations
- Jakpost Guide to
- Newsletter New
- Mobile Apps
- Tenggara Strategics
- B/NDL Studios
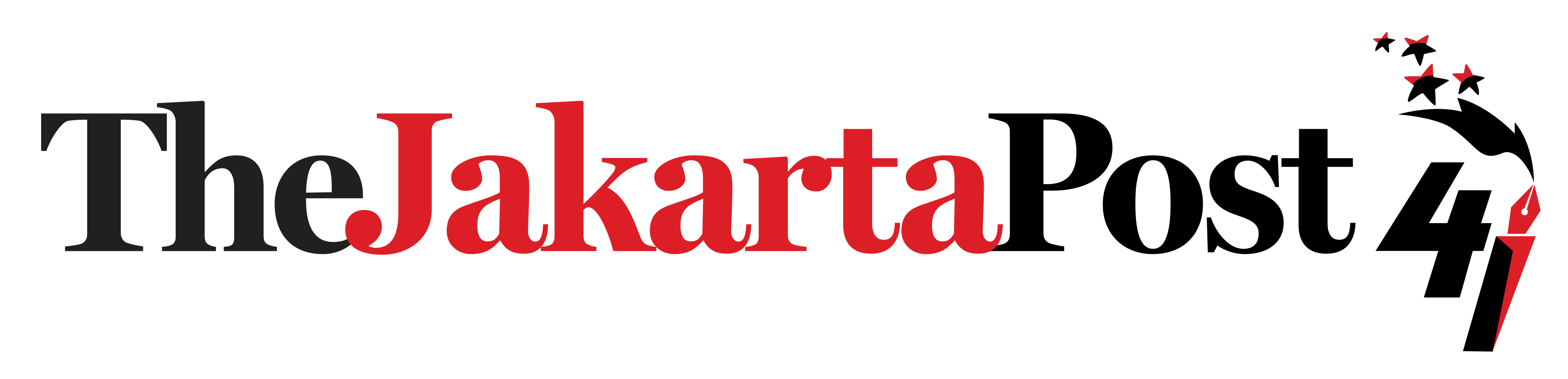
- Archipelago
- Election 2024
- Regulations
- Asia & Pacific
- Middle East & Africa
- Entertainment
- Arts & Culture
- Environment
- Work it Right
- Quick Dispatch
- Longform Biz
Dengue deaths spike almost threefold compared to last year
Share this article, change size.
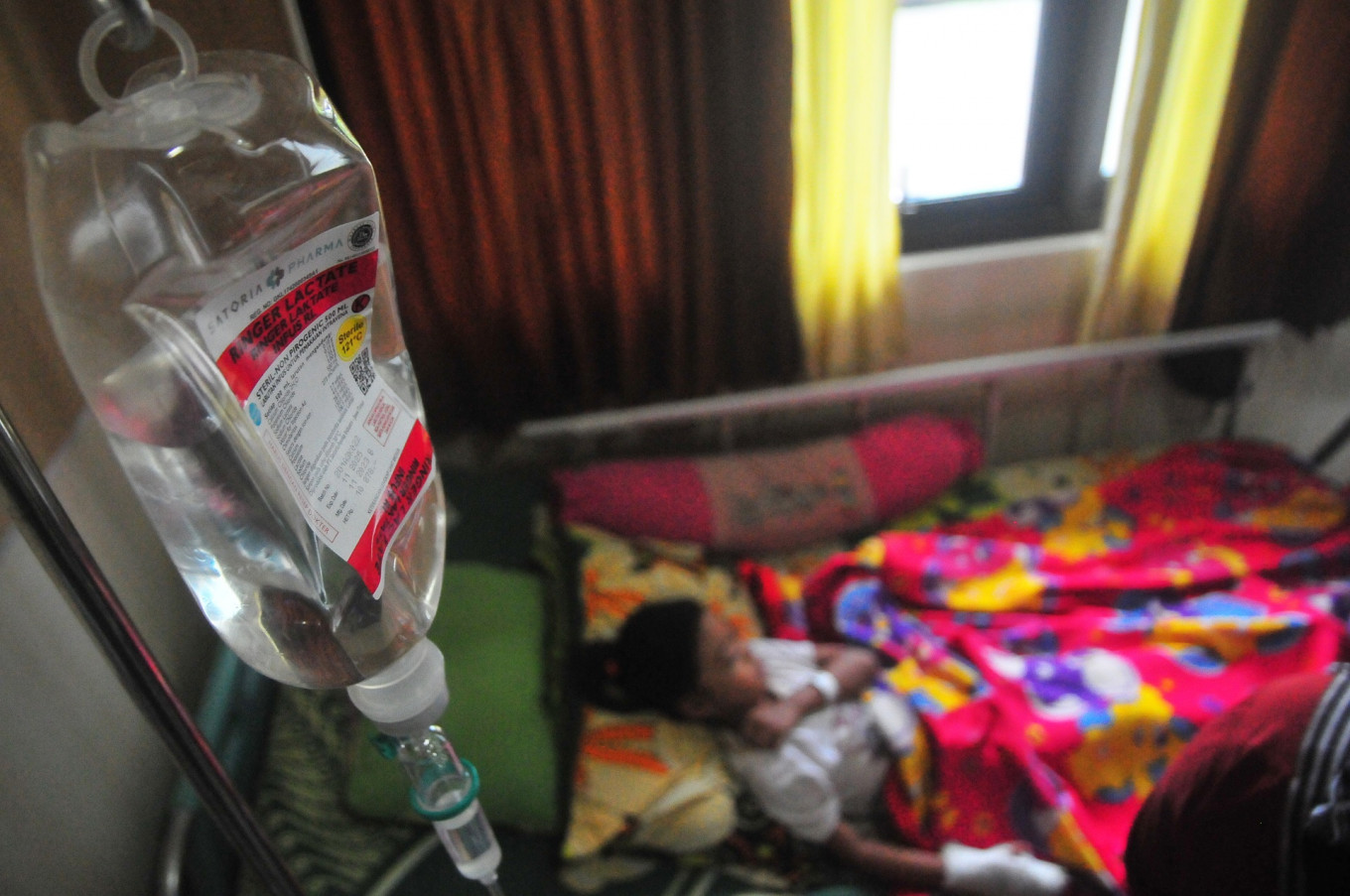
ndonesia has seen almost three times as many deaths from dengue fever so far this year compared to the same period last year, as warmer weather triggered by the El Niño climate phenomenon drives up cases across the country.
The Health Ministry has discovered roughly 62,000 dengue cases with 475 deaths from January to mid-April, almost triple the 22,500 cases and 170 deaths during the same period last year.
In January this year alone, Indonesia saw 25,218 cases of dengue, the highest monthly rate in five years. Dengue-related deaths reached 191 in February, the highest number of monthly fatalities since January 2022.
The ministry has blamed the recent outbreak on a warmer rainy season caused by the El Niño climate phenomenon, which speeds up mosquitoes' life cycles, helping them grow faster and live longer.
Warmer temperatures also speed up virus reproduction in infected mosquitoes and increase the frequency of mosquito bites, allowing them to spread diseases more quickly.
Read also: Community’s role crucial in protecting families against dengue

Morning Brief
Every monday, wednesday and friday morning..
Delivered straight to your inbox three times weekly, this curated briefing provides a concise overview of the day's most important issues, covering a wide range of topics from politics to culture and society.
By registering, you agree with The Jakarta Post 's Privacy Policy
for signing up our newsletter!
Please check your email for your newsletter subscription.
Dengue fever is a viral disease transmitted through the bite of infected Aedes aegypti and Aedes albopictus mosquitoes, which are commonly found in places with tropical climates such as Indonesia.
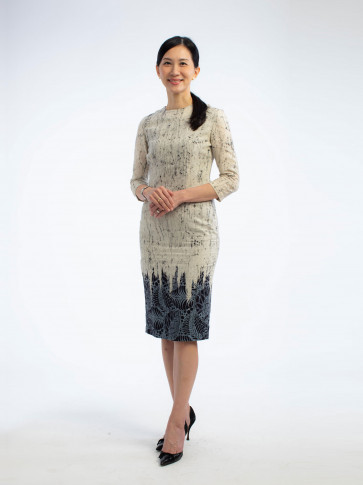
Women in philanthropy: Fifi Pangestu’s leadership and vision in Bakti Barito Foundation
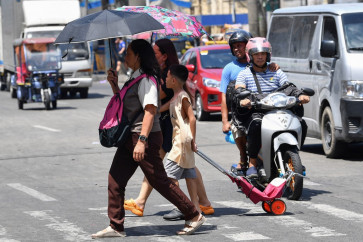
Philippines to endure extreme heat until mid-May
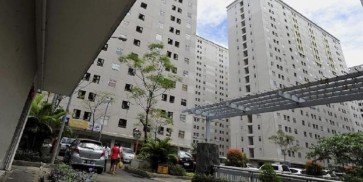
Magnitude 6.5 earthquake strikes off Java island: authorities
Related articles, dengue outbreak shows no signs of abating as toll rises, dawn of a worse dengue era, el nino has ended: australia's weather bureau, soaring coffee bean prices grind local business owners’ profits, indonesia braces for wildfires ahead of dry season, related article, more in indonesia.
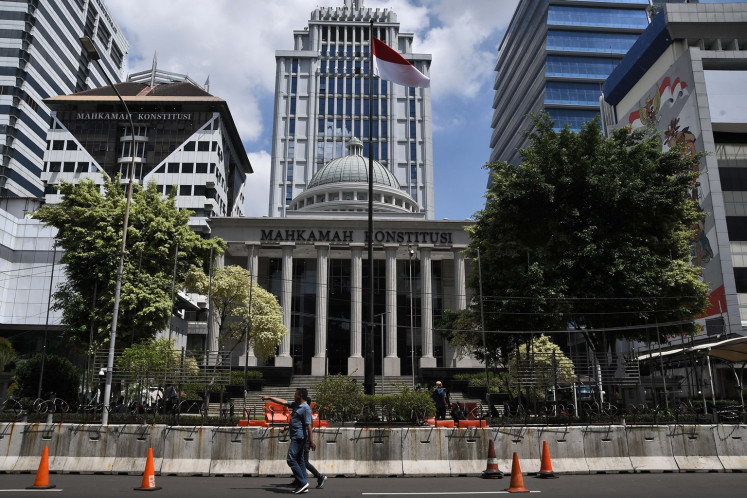
Court kicks off hearings for legislative election disputes
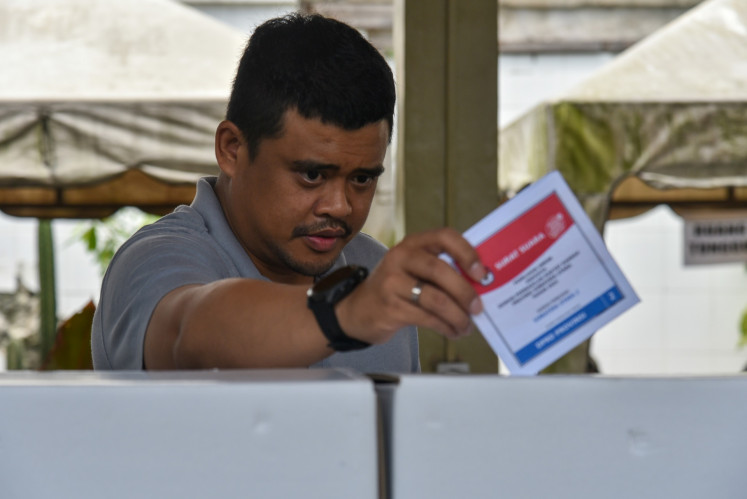
Golkar branch head up against Jokowi’s son-in-law for N. Sumatra governor
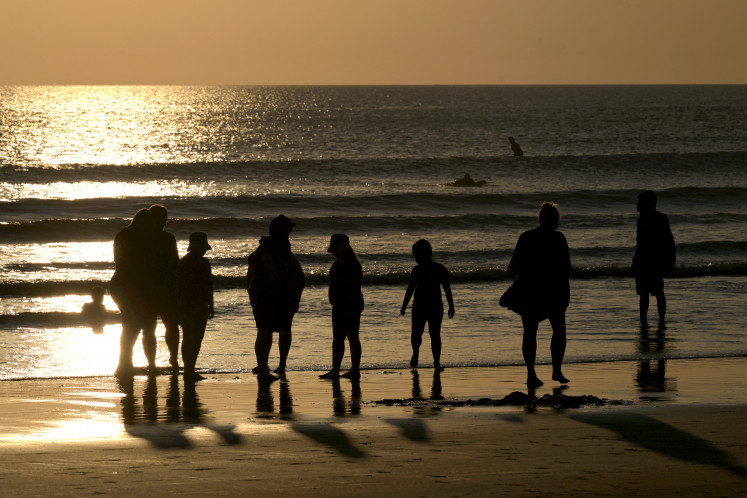
Korean nationals deported for illegal TV shoot in Bali
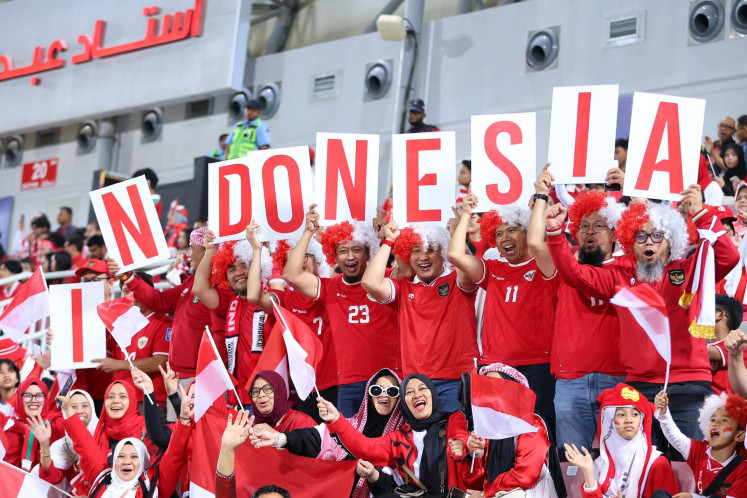
Indonesia wait for Olympic football goes on after Uzbekistan defeat
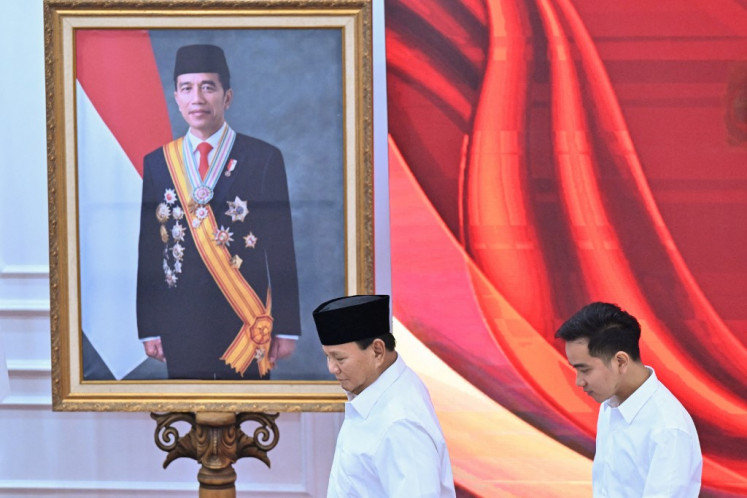
On letting go
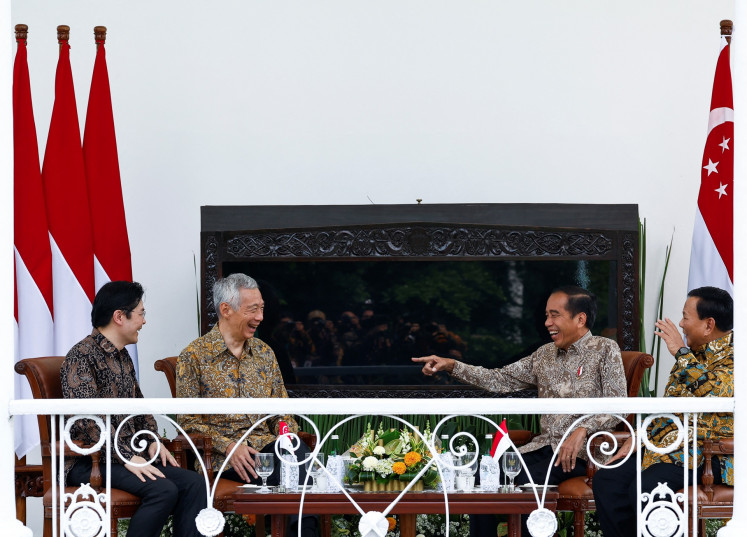
Jokowi, Lee in final retreat call on successors to maintain strong ties
Stocks in asia rise, yen wobbles after volatile start to week, plan for 70% renewables in 2024 ruptl could face challenges, goto says on track for profitability despite first-quarter loss, investment growth on track in first quarter, jump in f&b sector.

- Jakpost Guide To
- Art & Culture
- Today's Paper
- Southeast Asia
- Cyber Media Guidelines
- Paper Subscription
- Privacy Policy
- Discussion Guideline
- Term of Use
© 2016 - 2024 PT. Bina Media Tenggara
to Read Full Story
Subscribe now.
- Unlimited access to our web and app content
- e-Post daily digital newspaper
- No advertisements, no interruptions
- Privileged access to our events and programs
- Subscription to our newsletters
Purchase access to this article for
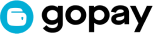
Redirecting you to payment page
Pay per article.
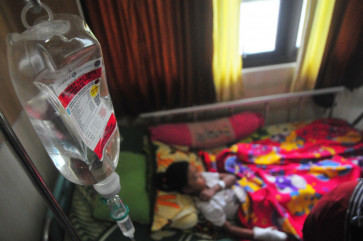
Rp 29,000 / article
Or continue login with
- Palmerat Barat No. 142-143
- Central Jakarta
- DKI Jakarta
- +6283816779933

Your Opinion Matters
Share your experiences, suggestions, and any issues you've encountered on The Jakarta Post. We're here to listen.
Thank you for sharing your thoughts. We appreciate your feedback.

An official website of the United States government
The .gov means it’s official. Federal government websites often end in .gov or .mil. Before sharing sensitive information, make sure you’re on a federal government site.
The site is secure. The https:// ensures that you are connecting to the official website and that any information you provide is encrypted and transmitted securely.
- Publications
- Account settings
Preview improvements coming to the PMC website in October 2024. Learn More or Try it out now .
- Advanced Search
- Journal List
- J Int Soc Prev Community Dent
- v.6(1); Jan-Feb 2016
Dengue virus: A global human threat: Review of literature
Shamimul hasan.
Department of Oral Medicine and Radiology, Jamia Millia Islamia, New Delhi, India
Sami Faisal Jamdar
1 Department of Oral and Maxillofacial Surgery, Teerthankar Mahaveer Dental College, Teerthanker Mahaveer University, Moradabad, Uttar Pradesh, India
Munther Alalowi
2 Buraydah College of Dentistry and Pharmacy, Al Qassim, Saudi Arabia
Sadun Mohammad Al Ageel Al Beaiji
3 Department of Conservative Dentistry and Endodontics, Specialist Dental Center, Hafer Al Batin, Saudia Arabia
Dengue is an acute viral illness caused by RNA virus of the family Flaviviridae and spread by Aedes mosquitoes. Presenting features may range from asymptomatic fever to dreaded complications such as hemorrhagic fever and shock. A cute-onset high fever, muscle and joint pain, myalgia, cutaneous rash, hemorrhagic episodes, and circulatory shock are the commonly seen symptoms. Oral manifestations are rare in dengue infection; however, some cases may have oral features as the only presenting manifestation. Early and accurate diagnosis is critical to reduce mortality. Although dengue virus infections are usually self-limiting, dengue infection has come up as a public health challenge in the tropical and subtropical nations. This article provide a detailed overview on dengue virus infections, varied clinical manifestations, diagnosis, differential diagnosis, and prevention and treatment.
INTRODUCTION
The dengue virus, a member of the genus Flavivirus of the family Flaviviridae, is an arthropode-borne virus that includes four different serotypes (DEN-1, DEN-2, DEN-3, and DEN-4).[ 1 , 2 ] The World Health Organization (WHO) consider dengue as a major global public health challenge in the tropic and subtropic nations. Dengue has seen a 30-fold upsurge worldwide between 1960 and 2010, due to increased population growth rate, global warming, unplanned urbanization, inefficient mosquito control, frequent air travel, and lack of health care facilities.[ 3 , 4 , 5 ] Two and a half billion people reside in dengue-endemic regions[ 5 ] and roughly 400 million infections occuring per year, with a mortality rate surpassing 5–20% in some areas.[ 6 ] Dengue infection affects more than 100 countries, including Europe and the United States (USA).[ 7 ] The first reported case of dengue like illness in india was in Madras in 1780, the first virologically proved epidemic of DF in India occurred in Calcutta and Eastern Coast of India in 1963-1964.[ 8 ] Dengue virus infection presents with a diverse clinical picture that ranges from asymptomatic illness to DF to the severe illness of dengue hemorrhagic fever/dengue shock syndrome (DHF/DSS).[ 4 ] Oral mucosal involvement is seen in approximately 30% of patients, although oral features are more frequently associated with DHF than with DF.[ 9 ] Dengue virus infection exhibit varied clinical presentation, hence, accurate diagnosis is difficult and relies on laboratory confirmation. The condition is usually self-limiting and antiviral therapy is not currently available. Supportive care with analgesics, hydration with fluid replacement, and sufficient bed rest forms the preferred management strategy.
ETIOPATHOGENESIS
DF is a severe flu-like infection that involves individuals of all age groups (infants, children, adolescents, and adults).[ 9 ] Transmission among human beings occurs by the mosquito Aedes aegypti and chiefly occurs during the rainy season.[ 10 ] The proposed etiologies for dengue virus infection are:
- Viral replication, primarily in macrophages[ 11 ]
- Direct skin infection by the virus[ 12 ]
- Immunological and chemical-mediated mechanism induced by host–viral interaction.[ 12 ]
Dengue virus gains entry into the host organism through the skin following an infected mosquito bite. Humoral, cellular, and innate host immune responses are implicated in the progression of the illness and the more severe clinical signs occur following the rapid clearance of the virus from the host organism. Hence, the most severe clinical presentation during the infection course does not correlate with a high viral load.[ 13 ] Alterations in endothelial microvascular permeability and thromboregulatory mechanisms lead to an increased loss of protein and plasma. Proposed theories suggest that endothelial cell activation caused by monocytes, T-cells, the complement system, and various inflammatory molecules mediate plasma leakage. Thrombocytopenia may be related to alterations in megakaryocytopoiesis, manifested by infection of human hematopoietic cells and compromised progenitor cell growth. This may cause platelet dysfunction, damage, or depletion, leading to significant hemorrhages.[ 14 , 15 ]
Figure 1 depicts a diagramatic representation of the pathogenesis of dengue.

Pathogenesis of dengue virus infection
CLASSIFICATION
The WHO classifies DF into two groups: Uncomplicated and severe.[ 16 , 17 ] Severe cases are linked to excessive hemorrhage, organ impairement, or severe plasma escape, and the remaining cases are considered uncomplicated.[ 17 ]
According to the 1997 classification, dengue can be divided into undifferentiated fever, DF, and DHF.[ 18 ] DHF was further subdivided into grades I–IV.
Grade I: Only mild bruising or a positive tourniquet test
Grade II: Spontaneous bleeding into the skin and elsewhere
Grade III: Clinical sign of shock
Grade IV: Severe shock - feeble pulse, and blood pressure cannot be recorded.[ 19 ]
Here, grades III and IV comprise DSS.[ 17 ]
CLINICAL MANIFESTATIONS
Undifferentiated fever.
This stage is seen mostly in the primary infection but may also occur following the initial secondary infection. Clinically, it is difficult to differentiate from numerous other viral diseases and often remains undiagnosed.
Dengue fever
DF follows both primary and secondary infections, and is most frequently encountered in adults and older children. Onset of symptoms is characterized by a biphasic, high-grade fever lasting for 3 days to 1 week.[ 20 , 21 ] Severe headache (mainly retrobulbar), lassitude, myalgia and painful joint, metallic taste, apetite loss, diarrhea, vomiting, and stomachache are the other reported manifestations. Dengue is also known as breakbone fever because of the associated myalgia and pain in joints.[ 16 , 22 ] Of patients with DF, 50–82% report with a peculiar cutaneous rash.[ 23 , 24 ] The initial rash is the result of capillary dilatation, and presents as a transient facial flushing erythema, typically occuring before or during the first 1–2 days of fever. The second rash is seen at 3 days to 1 week following the fever, and presents as a asymptomatic maculopapular or morbilliform eruption. Sometimes, individual lesions may merge and present as widespread confluent erythematous areas with pinpoint bleeding spots and rounded islands of sparing, giving a typical appearance of “white islands in a sea of red.”[ 23 , 25 ] The cutaneous rash is usually asymptomatic, and pruritis is reported only in 16-27% cases.[ 9 , 26 ] Bleeding episodes are infrequently seen in DF, although epistaxis and gingival bleeding, substantial menstruation, petechiae/purpura, and gastrointestinal tract (GIT) hemorrhage can occur.[ 20 , 27 ]
Dengue hemorrhagic fever
DHF is frequently seen during a secondary dengue infection. However, in infants it may also occur durring a primary infection due to maternally attained dengue antibodies.[ 28 ] The proposed diagnostic criteria for DHF includes:[ 29 ]
- a. Clinical parameters: Acute-onset febrile phase – high-grade fever lasting from 2 days to 1 week. Hemorrhagic episodes (at least one of the following forms): Petechiae, purpura, ecchymosis, epistaxis, gingival and mucosal bleeding, GIT or injection site, hematemesis and/or malena
Positive tourniquet and hepatomegaly.
- b. Laboratory parameters: Thrombocytopenia (platelet count <100,000/cu mm)
The hemorrhagic episodes in DHF are associated with multifactorial pathogenesis. Vasculopathy, deficiency and dysfunction of platelets and defects in the blood coagulation pathways are the attributed factors.[ 30 ] Decreased production of platelets[ 31 , 32 ] and increased destruction of platelets may result in thrombocytopenia in DHF.[ 33 ] The impaired platelet function causes the blood vessels to become fragile and this results in hemorrhage.[ 34 ]
The clinical course of DHF is characterized by three phases: Febrile, leakage, and convalescent phase. High-grade fever of acute onset along with constitutional signs and facial erythema characterizes the commencement of the febrile illness.[ 21 ] The initial febrile illness is marked by a morbilliform rash and hemorrhagic tendencies.[ 35 ] The fever persists for 2 days to 1 week and then drops to normal or subnormal levels when the patient either convalesces or advances to the plasma leakage phase.[ 36 ] High plasma escape cases are marked by frank shock with low pulse pressure, cyanosis, hepatomegaly, pleural and pericardial effusions, and ascites. Severe ecchymosis and gastrointestinal bleeding followed by epistaxis may also be noted in a few cases. Bradycardia, confluent petechial rashes, erythema, and pallor are seen during this phase.
Dengue shock syndrome
DSS is defined as DHF accompanied by a unstable pulse, narrow pulse pressure (<20 mmHg), restlessness, cold, clammy skin, and circumoral cyanosis. Progressively worsening shock, multiorgan damage, and disseminated intravascular coagulation account for a high mortality rate associated with DSS. The shock persists for a short span of time and the patient promptly recovers with supportive therapy.[ 37 , 38 ]
OROFACIAL FEATURES
Oral features are infrequently seen in dengue virus infection and are more commonly associated with DHF. Erythema, crusting of lips, and ntongue and soft palatal vesicles constitute the prominent oral features in dengue virus infection. Chadwick et al .[ 26 ] reported higher cases involving the mucosa with scleral injection (90%), whereas Sanford noticed vesicular eruptions of the soft palate (>50%).[ 39 ] Byatnal et al ., reported numerous hemorrhagic bullae on the sublingual mucous membrane, lateral surface of the tongue, and floor of the mouth. Brown-colored plaquelike lesions with a rough surface were seen on the buccal mucosa that showed bleeding on touch along with spontaneous bleeding from the gingiva and the tongue. Petechiae, purpura, ecchymoses, and nasal bleeding have also been reported.[ 40 ] Mitra et al . reported bleeding gums, hemorrhagic plaques, and inflamed tonsils in a dengue-infected patient.[ 41 ] Isolated hypoglossal nerve palsy following dengue infection is a rare occurence.[ 42 ] Taste alteration, conjunctival redness, and lymphadenopathy may also be reported in DF.[ 3 ] Table 1 depicts the reported orofacial features of dengue.
Summary of reported orofacial features in dengue[ 4 ]

Cautious attention should be directed at DF if a patient suffers from high fever within 2 weeks of being in the tropics or subtropics.[ 43 ] A decreased number of white blood cells (leukopenia), accompanied by a decreased number of platelet count (thrombocytopenia) and metabolic acidosis are the initial changes on laboratory examinations. Microbiological laboratory testing confirms the diagnosis of DF. Virus segregation in cell cultures, nucleic acid demonstration by polymerase chain reaction (PCR), and serological detection of viral antigens (such as NS1) or particular antibodies are the preferred microbiological assays.[ 5 ] Viral segregation and nucleic acid demonstration provide precise diagnosis, although the high cost limits the availability of these tests.
DIFFERENTIAL DIAGNOSIS
Broad differential diagnosis is considered in a patient presenting with fever and a rash similar to that seen in DF. Tables Tables2 2 and and3 3 present the varied clinical conditions that mimic the febrile and critical phase of dengue infection.
Conditions that mimic the febrile phase of dengue infection[ 4 ]

Conditions that mimic the critical phase of dengue infection[ 4 ]

MANAGEMENT OF DENGUE INFECTION
Fluid replacement and antipyretic therapy with paracetamol is the preferred therapy following the febrile phase. Care should be taken not to use other nonsteroidal antiinflammatory drugs.
Judicious fluid administration forms the mainstay of treatment during the critical phase of the infection. Normal saline, Ringer's Lactate, and 5% glucose diluted 1:2 or 1:1 in normal saline, plasma, plasma substitutes, or 5% albumin are the routinely administered fluids.
WHO guidelines summarize the following principles of fluid therapy:[ 44 ]
- Oral fluid supplementation must be as plentiful as possible. However, intravenous fluid administration is mandatory in cases of shock, severe vomiting, and prostration (cases where the patient is unable to take fluids orally)
- Crystalloids form the first-line choice of intravenous fluid (0.9% saline)
- Hypotensive states that are unresponsive to boluses of intravenous crystalloids, colloids (e.g., dextran) form the second-line measures
- If the patient remains in the critical phase with low platelet counts, there should be a serious concern for bleeding. Suspected cases of bleeding are best managed by transfusion of fresh whole blood.
DENTAL MANAGEMENT
Oral lesions are infrequently seen and are often misguided as platelet defects.[ 25 ] Significant hemorrhagic manifestations need platelet transfusions. In general, there is no need to give prophylactic platelets even at <20,000/cu mm. Prophylactic platelets may be given at a level of <10,000/cu mm in absence of bleeding manifestations. In case of systemic massive bleeding, platelet transfusion may be needed along with red cell transfusion. Liver functions should be monitored.
ADVANCED RESEARCHES
Control of mosquito (vector) transmission, development of dengue vaccine, and antiviral drugs constitute future directions with an aim to prevent and treat dengue infection.
Control of mosquito (vector) transmission can be done by keeping guppies ( Poecilia reticulata ) or copepods ( doridicola agilis ) in standing water, and infecting the mosquito population with bacteria of the Wolbachia genus.[ 43 ]
Due to the progressing transmission and enhancing severity of dengue infection, the necessity to develop a dengue vaccine has gained considerable importance. There is a worldwide public health need for a safe, effective, and economic tetravalent dengue vaccine. Complex pathology, the prerequisite to control four virus serotypes, and inadequate investment by vaccine designers have hindered vaccine advancement.[ 45 ]
Scrupulous attempts are aimed to develop antiviral drugs that can be used to manage DF and avoid the life-threatening episodes.[ 46 , 47 ]
Dengue has evolved as a global life-threatening public health concern, affecting around 2.5 billion individuals in more than 100 countries. The physician should be aware about the varied clinical manifestations of this condition and ensure an early and adequate treatment plan. Future directions to combat this dreadful disease aim at methods of mosquito control, development of vaccine, and antiviral drug regimen.
Financial support and sponsorship
Conflicts of interest.
There are no conflicts of interest.
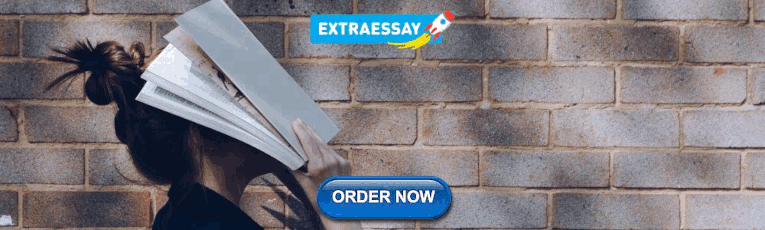
COMMENTS
In November 2009, WHO issued a new guideline that classifies symptomatic cases as dengue or severe dengue. Dengue is defined by a combination of ≥2 clinical findings in a febrile person who traveled to or lives in a dengue-endemic area. Clinical findings include nausea, vomiting, rash, aches and pains, a positive tourniquet test, leukopenia, and the following warning signs: abdominal pain or ...
Abstract. Dengue is the major cause of arthropod-borne viral disease in the world. It presents with high fever, headache, rash, myalgia, and arthralgia and it is a self-limiting illness. Severe dengue can occur in some cases resulting in dengue hemorrhagic fever (DHF) and dengue shock syndrome (DSS). We present a case of a 32-year-old male ...
Case presentation. Case 1. A 37-year-old female homemaker, with no comorbidities, presented to the emergency department with altered mentation. ... Dengue virus was earlier considered non-neurotropic, but recent observations have proven this wrong. Direct viral invasion in the CNS, possibly as a result of the blood brain barrier disruption, is ...
Dengue (den-gee) Fever (DF) is a mosquito-borne disease caused by the dengue virus. The virus belongs to the family Flaviviridae and genus flavivirus.1 Like other flaviviruses, such. as yellow fever virus and West Nile virus, the dengue virus utilizes single stranded RNA as its. genetic content.1 The disease is found primarily in tropical and ...
Atypical manifestations are rare and include encephalopathy, encephalitis, seizures, hepatocellular damage, acalculous cholecystitis, myocarditis, pericardial effusion, and severe gastrointestinal hemorrhage.[6,7] The clinical presentation in dengue depends on the virus strain, as well as the age and immune status of the host. This study aims ...
Case lessons •The DDx of returned febrile travelers is broad, even if the presentation fits a specific diagnosis well •Misdiagnosis or no consideration of travel related infections are common at front-line healthcare settings in the US •Post-pandemic travel has resulted in increased numbers of dengue in our clinic, including from Asia and ...
The highest number of dengue cases was recorded in 2023, affecting over 80 countries in all regions of WHO. ... The dengue virus is transmitted to humans through the bites of infected female mosquitoes, ... J.J., et al., Viremia and Clinical Presentation in Nicaraguan Patients Infected With Zika Virus, Chikungunya Virus, and Dengue Virus ...
An interesting feature was that fever was a late clinical presentation in cases presenting with co-infection as compared to monoinfection. ... Pardo L, Rico A, Campo A, et al Clinical and histopathological features of fatal cases with dengue and chikungunya virus co-infection in Colombia, 2014 to 2015 Euro Surveill. 2016;21 doi: 10.2807/1560 ...
Dengue Case Management Dengue is the fastest-growing arborvirus infection with a rapidly evolving epidemiology. During the past 50 years, the worldwide incidence of dengue has risen 30-fold. The World Health Organization estimates that 50 100 million dengue infections occur each year in
Case presentation. Case 1 was a 39-year old Asian male, presented on day 6 of dengue infection with warning signs. Reverse transcription polymerase chain reaction for severe acute respiratory syndrome coronavirus 2 that was done as per hospital protocol was found to be positive. ... Two cases of dengue virus type 2 (DENV-2) infection in a ...
case series illustrates the non-specific nature and unpredictability of dengue fever, and attempts to clarify the management dilemma when dealing with presentations on both ends of the spectrum. 2. Case Presentation . 2.1. Case 1 (Group A) A 19 year-old Malaysian Indian male presented to the emergency department with two days of high fever
We report an atypical and rare presentation of dengue disease marked by a dramatic and fatal cardiogenic shock due to acute myocarditis. Histopathological analysis of heart tissue showed several multifocal areas of muscle necrosis and intense interstitial oedema associated with clusters of virus particles inside the cardiomyocytes and in the ...
Multifaceted Pre sentations of Dengue Virus Infecti on. and Its Management: A Case Series. Rafiq Shajahan 1,*, Puay Sheng Hwa, Choe Yee Xian, Naganathan Pillai, Ganesh Kasinathan. 1 Department of ...
Dengue cases have increased in the Americas over the past four decades, from 1.5 million cases from 1980 to 1989 to 17.5 million in 2010-2019. Before 2023, the highest historical dengue caseload was in 2019, with over 3.18 million cases, 28 208 severe cases, and 1823 deaths (CFR 0.06). ... Dengue virus (DENV) is transmitted to humans through ...
Dengue viruses are transmitted by the bite of the Aedes aegypti mosquito infected by the one of the four dengue virus serotypes: dengue-1, -2, -3, and -4. More recently, dengue disease has spread geographically to many previously unaffected areas and, as travelling around the world has become more accessible, physicians in temperate areas are ...
Dengue is a mosquito-transmitted virus and the leading cause of arthropod-borne viral disease in the world. It is also known as breakbone fever due to the severity of muscle spasms and joint pain, dandy fever, or seven-day fever because of the usual duration of symptoms. Although most cases are asymptomatic, severe illness and death may occur. Aedes mosquitoes transmit the virus and are common ...
Dengue is the most prevalent mosquito-borne viral disease in humans, and cases are continuing to rise globally. In particular, islands in the Caribbean have experienced more frequent outbreaks ...
A dengue vaccine is approved for use in children aged 9 to 16 years with laboratory-confirmed previous dengue virus infection and living in areas where dengue is endemic (common). Endemic areas include some U.S. territories and freely associated states. The vaccine is not approved for use in U.S. travelers who are visiting but not living in an ...
Case Presentation Dengue Fever - Download as a PDF or view online for free. ... Replication and Transmission of Dengue Virus (Part 1) 1. Virus transmitted to human in mosquito saliva 2. Virus replicates in target organs 3. Virus infects white blood cells and lymphatic tissues 4. Virus released and circulates in blood 3 4 1 2
Dengue fever (DF) is an emerging arboviral disease caused by the dengue virus (DENV) and is endemic in tropical and subtropical countries worldwide. 1 The incidence of DENV infections was estimated to be almost 400 million per year, of which about 25% were clinically apparent decades ago. 2 The reported cases of dengue have increased ...
Dengue viruses (DENV) are positive-stranded RNA viruses belonging to the Flaviviridae family. DENV is the causative agent of dengue, the most rapidly spreading viral disease transmitted by mosquitoes. Each year, millions of people contract the virus through bites from infected female mosquitoes of the Aedes species. In the majority of individuals, the infection is asymptomatic, and the immune ...
Dengue is an acute mosquito-borne illness that is caused by four different dengue viruses (serotypes 1-4). More than half the world's population lives at risk of dengue. ... Download .PPT; Linked Articles. Efficacy and immunogenicity following dengue virus-1 human challenge after a tetravalent prime-boost dengue vaccine regimen: an open-label ...
Update: April 18, 2024 14:00 PM (GMT-5)Between epidemiological weeks (EW) 1 and 13 of 2024, a total of 4,820,955 suspected cases of dengue were reported, resulting in a cumulative incidence of 511 per 100,000 population. This figure represents an increase of 260% compared to the same period in 2023 and 448% compared to the average of the last 5 years. Figure 1 shows the trend of suspected ...
The World Health Organization (WHO) listed DF as one of the top ten global health risks. The dengue virus infects an estimated 390 million people each year, with 96 million showing clinical symptoms. In the previous decade, the number of cases in Southeast Asia has grown . In tropical and sub-tropical nations, DF is a public health issue.
Dengue cases are surging in the Americas, with cases reported topping 5.2 million as of this week, surpassing a yearly record set in 2023, according to the Pan American Health Organization (PAHO).
PAHO, a United Nations agency, has confirmed more than 5.2 million cases of dengue across the Americas this year, an over 48% jump from the 3.5 million cases the group reported late last month.
Mosquitoes spread the virus by biting an infected person and spreading it to another person through their bites. French health authorities warn of the risks of imported cases of dengue fever ahead ...
The Health Ministry has discovered roughly 62,000 dengue cases with 475 deaths from January to mid-April, almost triple the 22,500 cases and 170 deaths during the same period last year.
The dengue virus, a member of the ... The first reported case of dengue like illness in india was in Madras in 1780, ... Dengue virus infection exhibit varied clinical presentation, hence, accurate diagnosis is difficult and relies on laboratory confirmation. The condition is usually self-limiting and antiviral therapy is not currently available.